The 4 big black hole frontiers for gravitational waves
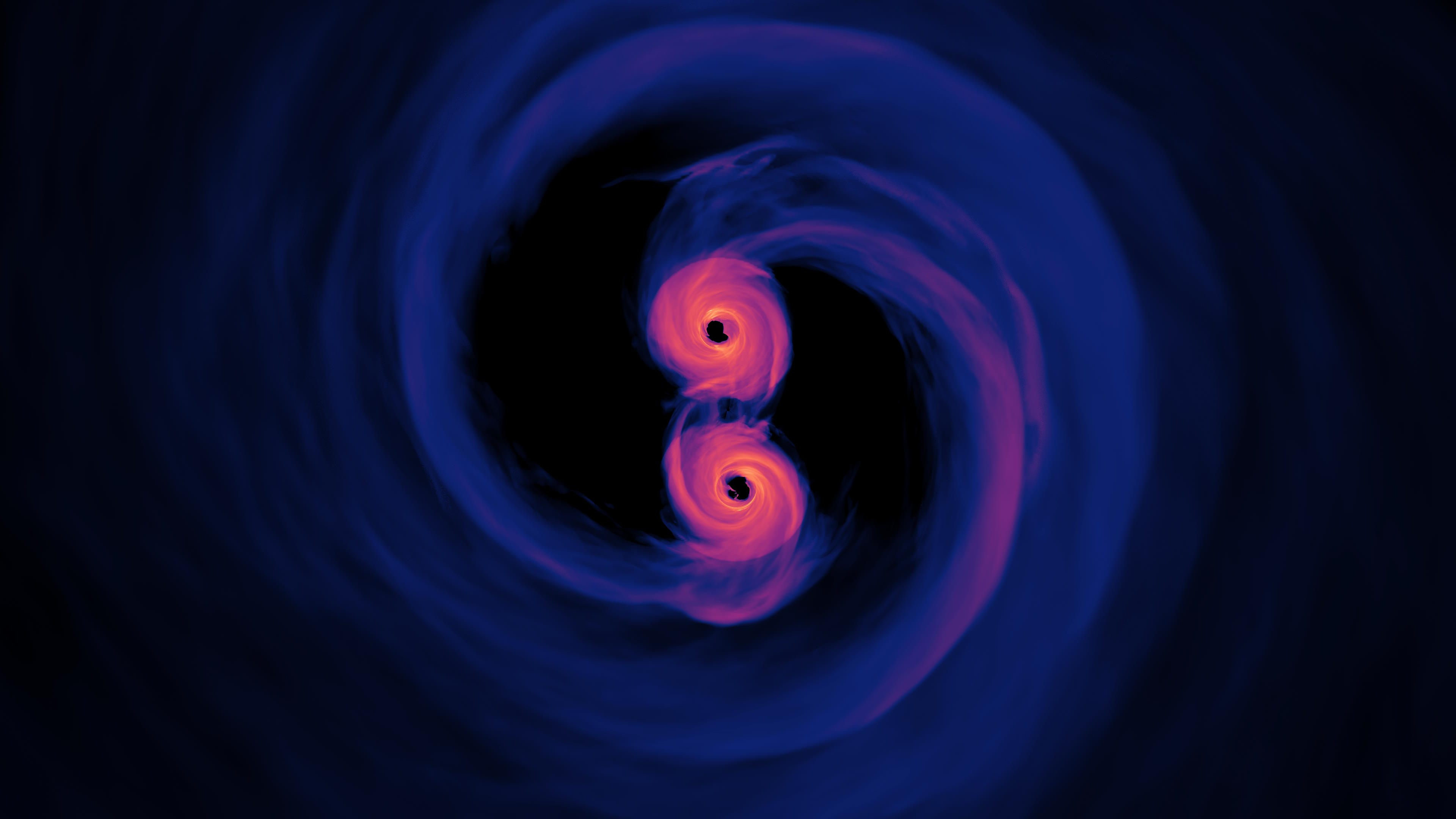
- Starting in 2015 with advanced LIGO, gravitational waves have revolutionized how we find and measure the black holes and neutron stars within our Universe.
- Last year, in 2023, the first strong evidence for a new type of gravitational wave signal, a cosmic gravitational wave background, was revealed by pulsar timing measurements.
- The ultimate goal is a complete census and understanding of all the black holes in the Universe, from the lowest-mass ones to the grandest cosmic behemoths. Here's how we're going to get there.
It’s hard to believe, but less than 10 years ago, humanity still wasn’t certain that gravitational waves were real. Sure, we had seen evidence that massive objects in tight orbits were exhibiting orbital decay, but we had yet to directly detect the energy being radiated away from them: theoretically in the form of gravitational waves. All of that changed with the dawn of Advanced LIGO, which detected its first gravitational wave signal — from two merging black holes — on September 14, 2015. In the time since, LIGO has been upgraded, joined by additional gravitational wave detectors, and has seen over 100 mergers and merger candidates, detecting low-mass neutron stars and black holes up to ~100 times the mass of our Sun.
Then, last year, in 2023, a novel method for detecting gravitational waves achieved its first success. By using an array of natural, astronomical clocks — millisecond pulsars — astronomers were able to uncover the first evidence for a low-frequency gravitational wave background to the Universe. The ultimate goal of gravitational wave astronomy is ambitious: a complete census and knowledge of the black hole and neutron star populations that are out there, as well as the event rates for inspirals and mergers throughout cosmic history. Remarkably, there are four frontiers that are actively being pushed back by gravitational wave advances. Here’s what’s coming up to improve our understanding of the Universe.
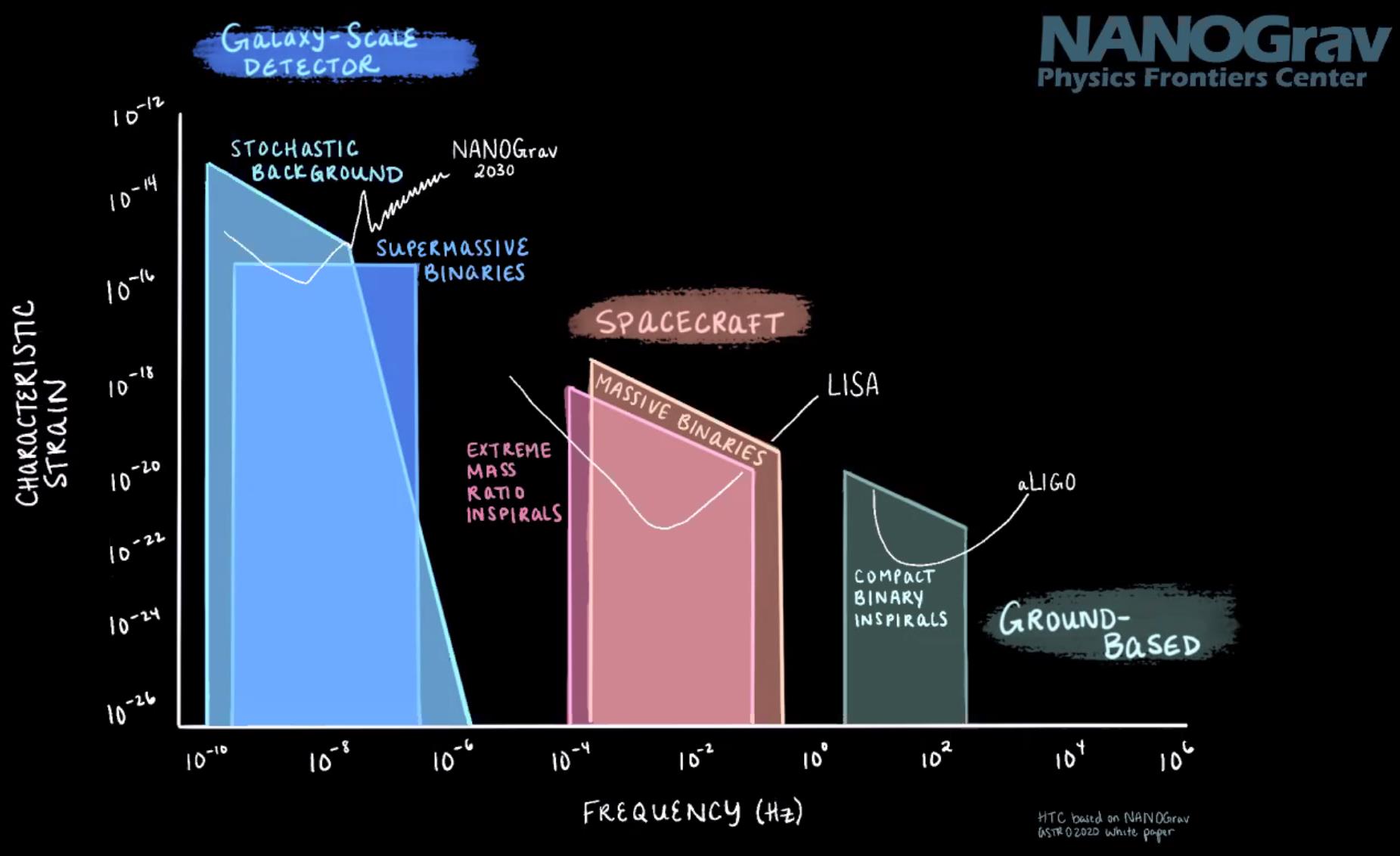
1.) The most massive black holes of all.
One of the most remarkable aspects of gravitational waves is that they are generated whenever you have:
- one mass,
- in an accelerated motion,
- through a region of curved space,
- caused by the presence of another mass.
This applies to pretty much all gravitating systems, from the Earth-Moon system to our Solar System to the stars in the galaxy and all the galaxies in a group or cluster of galaxies. However, if you want the strongest gravitational wave signals, you’ll look for the largest masses at the closest orbital distances possible to one another.
This means that the gravitational waves that exhibit the largest amplitudes will come from the most massive orbiting systems where the two masses achieve the closest distances possible to one another: from binary supermassive or ultramassive black holes, especially in the moments just before their two event horizons touch one another. The combined effects of all the supermassive and ultramassive black holes together, all throughout the Universe, is the expected and most likely culprit behind the background of gravitational waves detected through pulsar timing. With incremental improvements that are already in progress, it’s fully expected that the first individual systems — the closest-orbiting and most massive ones — will soon emerge from within this data.
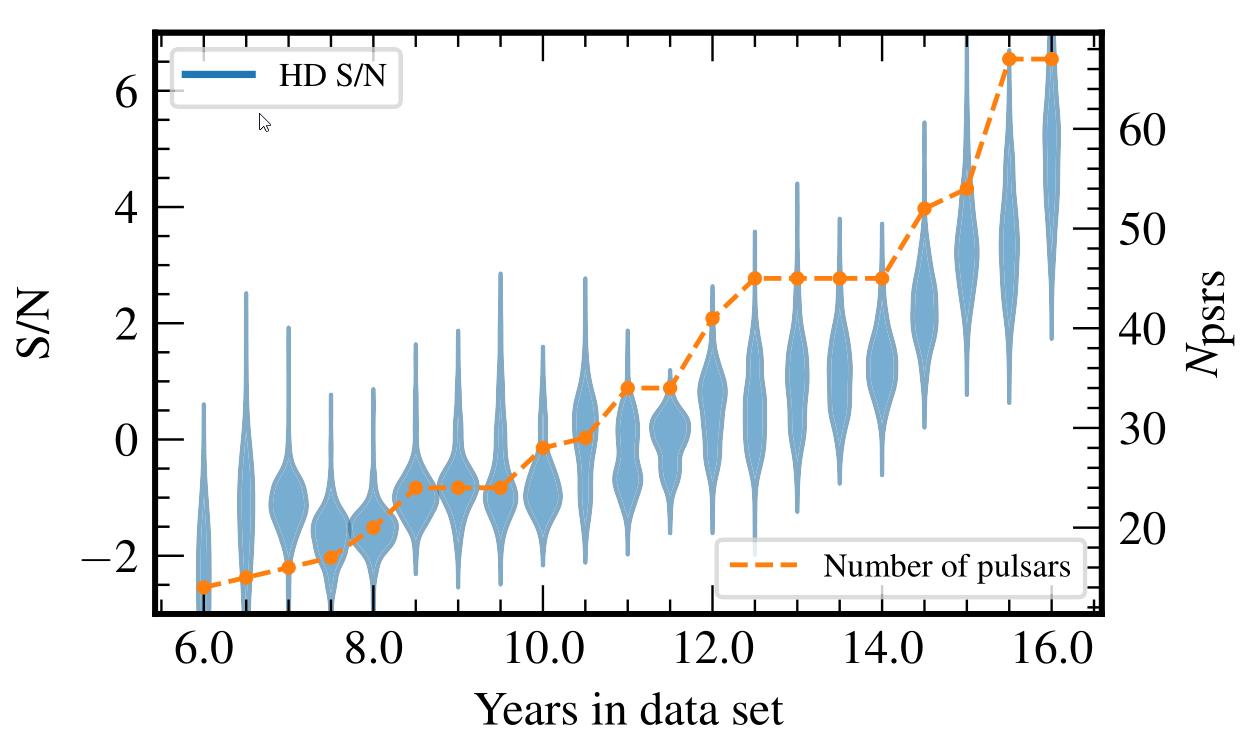
If you had just a single millisecond pulsar whose timing you could measure perfectly, you would measure the combined effects of all of the gravitational waves that passed through it, shortening-and-lengthening the distance that the light traveled on its journey from the pulsar to our telescopes in a regular and predictable fashion. Although individual pulsars (and the telescopes and arrays that monitor them) all have errors and uncertainties inherent to them, a large array of pulsars is much better. Especially if your system is relatively stable over long timescales (which is expected for the most massive systems), those gravitational wave signals will wash over all of your pulsars at once, allowing a strong enough individual signal to be picked out.
If there are two black holes, each of a billion solar masses or more, orbiting one another at very close distances (where they may merge in the next few million years) almost anywhere within the observable Universe, pulsar timing measurements should be able to pick out these individual objects at some point in the next decade or two. This is a rapidly advancing field, and with the combination of existing facilities and upcoming new observatories — such as the proposed ngVLA (next-generation Very Large Array) — these ultramassive black hole binaries, which ought to lead to the most massive black holes in all the Universe, will soon be revealed.
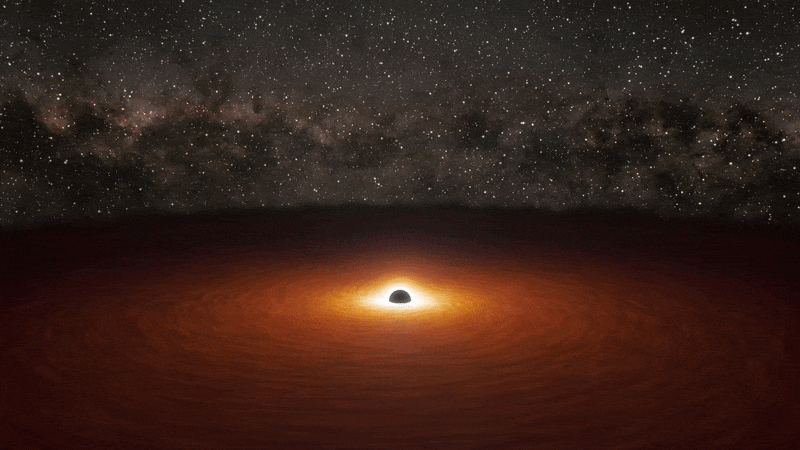
2.) Supermassive black holes and extreme mass-ratio mergers.
Although the ultramassive black holes in the Universe — the most massive black holes of all, in the billions to tens of billions of solar masses — might be far and away the most impressive, they don’t represent the majority of supermassive black holes. At the centers of nearly all known galaxies lie supermassive black holes that are relatively more modest: possessing millions, tens of millions, or perhaps hundreds of millions of solar masses, such as the black holes at the centers of the Milky Way, Andromeda, and the majority of known, large galaxies. Because of their lower masses, however, pulsar timing is likely a very long way away from being sensitive to their presence, even if they’re in a binary system, possessing another orbiting black hole companion.
Meanwhile, we cannot hope to detect these objects using ground-based detectors, as objects orbiting a ~million solar mass black hole will emit gravitational waves with a characteristic period of about 100-to-1000 seconds, whereas LIGO and other terrestrial detectors can only detect gravitational waves emitted with a period between milliseconds and a few tenths of a second. Fortunately, there’s a solution that isn’t just theoretical, but that is being designed and built to fly in just a few years: a space-based gravitational wave detector with enormous distances separating the laser arms. The version that’s going to fly is called LISA: the Laser Interferometer Space Antenna.

As these three spacecraft fly through space, the distance between them changes over time in a predictable fashion, but with the gravitational wave signals that appear as ripples in spacetime superimposed atop them. For the first time, black holes that are more massive than terrestrial detectors are sensitive to (up to perhaps a few hundred solar masses) but less massive than the billion-ish solar mass behemoths (and up) that pulsar timing is sensitive to will fall within our reach. Out to billions of light-years away, LISA will be sensitive to:
- binary black holes (of comparable masses) weighing in from around 10,000 to tens of millions of solar masses,
- extreme mass ratio inspirals, where objects of only ~1-100 solar masses fall into a typical supermassive black hole,
- and the types of black holes that LIGO and other ground-based detectors are sensitive to, except during a very early stage of the inspiral phase, enabling us to predict when (and, quite possibly, where) a stellar mass binary black hole merger will occur.
It’s important to remember that even though LIGO has detected an enormous number of black holes, and is increasingly becoming more and more sensitive to black holes and neutron stars at greater distances, the most massive black hole it’s ever seen is barely over 100 solar masses. There remains an incredibly important population of black holes at the centers of galaxies that has only been detected largely indirectly, through the luminous radiation emitted by objects around them. Seeing them in gravitational waves would, and likely will, open up a whole new window onto the Universe.

3.) Intermediate mass black holes and black holes beyond stellar production.
Even when LISA is operating in space and advanced LIGO (plus Virgo, KAGRA, and LIGO India) operate on the ground, there’s still going to be a gap between the highest-mass black hole that ground-based detectors can see, capping out at around ~200 solar masses, and the lowest-mass black hole that a space-based detector can see, down to about ~10,000 solar masses.
What about the population that lies in this in-between range: beyond what even the most massive stars can produce, but only by a relatively small amount?
There is a way to get there: by building an ultra-advanced ground-based gravitational wave detector that’s about ten times the size of the LIGO detectors that exist today. Remember that today’s ground-based gravitational wave detectors work by:
- splitting light and firing it down long, straight, perpendicular arms,
- with mirrors at both ends,
- that reflect that light back-and-forth about 1000 times,
- before bringing that light back together,
- and looking at how the interference pattern it makes shifts over time,
whereby we can extract any gravitational wave signal of the right frequency to cause that interference pattern to shift.

With ~4 kilometer arms, LIGO is sensitive to gravitational waves generated with a period of about 1 second or less. But if it had ~40 kilometer arms instead, it could detect gravitational waves that take longer to generate: from black holes with larger event horizons and greater masses than the current generation of gravitational wave detectors can see.
Of course, there are numerous challenges to building such a large gravitational wave observatory. You’d have to reckon with:
- the fact that these arms would have to be perfectly straight, requiring that either tunnels be built underground or large support structures above-ground to account for the curvature of the Earth,
- that literally ten times the amount of land would be needed, as finding a suitable “L” shaped piece of land that’s available for 40 kilometers in two perpendicular directions is challenging,
- and that the will to publicly fund large-scale projects like this is in constant jeopardy in the 21st century’s austere political climate,
among other issues. But these are not fundamental science issues. Advances in squeezed quantum states for laser light, in seismic noise floor reductions for current gravitational wave detectors, and in handling the enormous amounts of data and auto-searching them, rapidly, for candidate events all help make the science case for the next generation of ground-based gravitational wave detectors. If we build them, we’ll at last be able to understand how black hole mergers allow them to grow from stellar mass to supermassive varieties.

4.) The lightest black holes of all and the so-called “mass gap.”
There’s also a very big question that still stumps the best astrophysicists studying the Universe today: where is the line between the heaviest neutron star and the lightest black hole, and how are those objects formed? We know a few things already.
- We’ve seen black holes with masses as low as just over 3 solar masses, and neutron stars that are as heavy as just over 2 solar masses.
- Observationally, we know that if two neutron stars of a low enough combined mass merge together, they’ll create a neutron star; if their combined mass is high enough, they’ll create a black hole.
- And from the closest gravitational wave event ever seen, 2017’s neutron star-neutron star merger just 130 million light-years away, we can be fairly certain that we’ve seen a neutron star-neutron star merger briefly become a neutron star for a fraction of a second, before collapsing into a black hole.
The prevailing wisdom is that both the heaviest neutron stars and the lightest black holes are created from neutron star-neutron star mergers, and very recently, the science of pulsar timing has just revealed that one particular pulsar, PSR J0514-4002E, has a binary companion that is a compact object whose mass is between 2.09 and 2.71 solar masses, making it either the heaviest neutron star or the lightest black hole known.

This is important, because the main way that black holes are created in the Universe — from the collapse of the core of a massive star — struggles to produce black holes below about 5 solar masses. Originally, that “2 to 5 solar mass” range was known as the mass gap, as there was a long period of time where there were practically no objects found in that range. At present, however, we know there are neutron stars above 2 solar masses and that, once you get above perhaps 2.7 or 2.8 solar masses, everything needs to be a black hole. (It’s thought that, at the high-mass end, you can have neutron stars or black holes, dependent on the object’s spin.)
Probing this regime is fascinating, because it’s perhaps the only case where both pulsar timing and ground-based gravitational wave detectors are sensitive to the same class of object. If you have a merging set of neutron stars, they can give rise either to a neutron star or black hole, while if you have a pulsing neutron star in orbit around a binary companion, that companion can:
- be a black hole,
- or be a neutron star,
- or be a full-fledged massive star that will reach the end of its life and die, becoming either a black hole or neutron star.
It is not yet known which method will be more informative for probing this borderline region, at the edge of where neutron stars and black holes are possible, but both classes of observations will be vital to solving this puzzle.
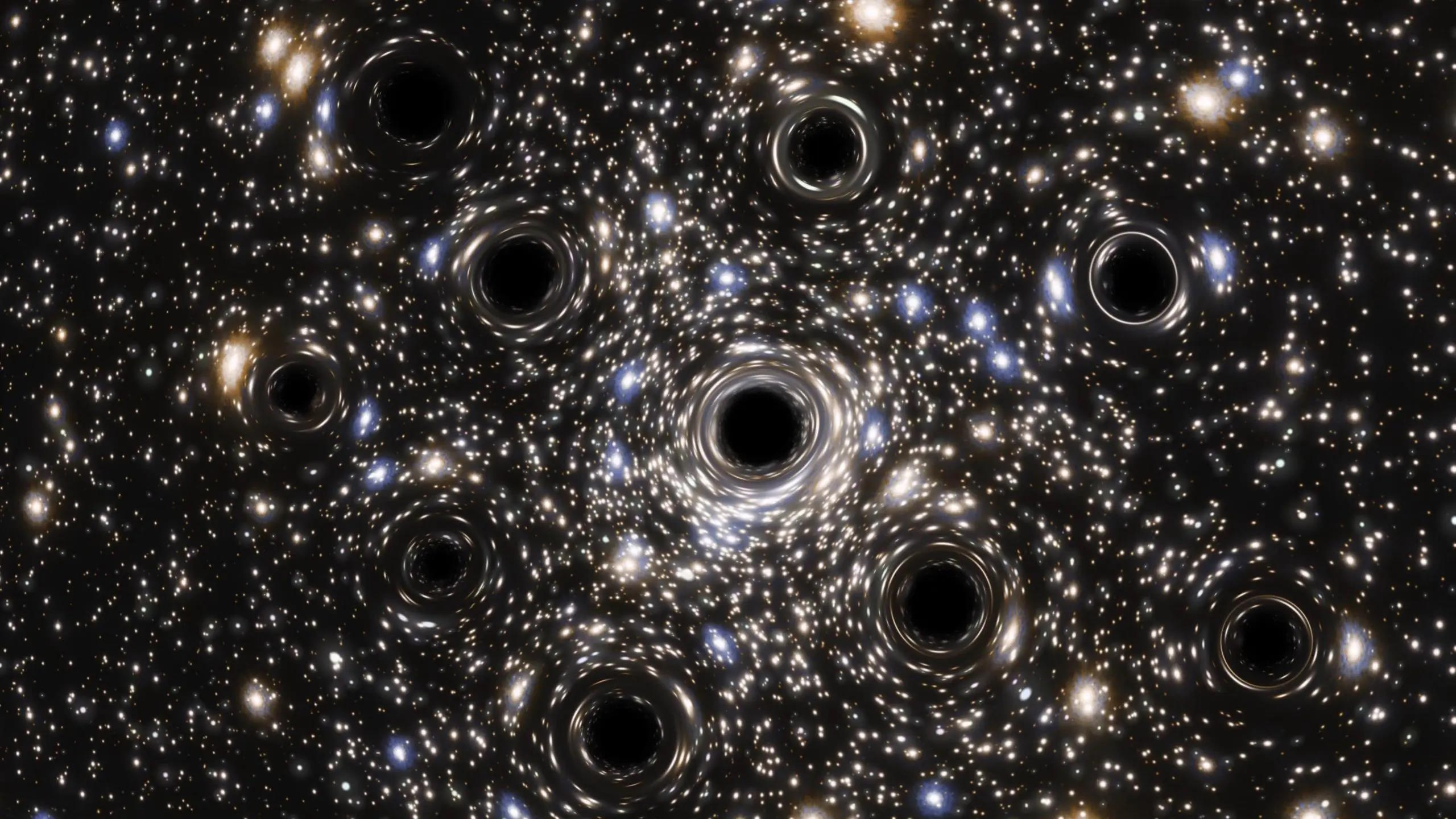
It was only a few years ago, in the early 21st century, where the only evidence we had for black holes was indirect: from the electromagnetic emissions that occurred from matter being accelerated around the outside of the black hole, or from stars that appeared to orbit a mysterious, massive point where no light appeared to emerge. Today, all of that has changed tremendously, as in addition to those lines of evidence, we now have:
- direct images of the event horizons around black holes,
- gravitational wave detections, from pulsar timing, of a stochastic background of gravitational waves permeating the Universe,
- and gravitational wave detections, from inspiraling and merging compact objects, that reveal neutron star and black hole mergers from ~1 up to ~200 solar masses.
The ultimate goal is to be able to characterize the entire population of black holes, of all masses, all throughout the Universe. We already have an estimate for the total number and total mass of all black holes in the observable Universe, combined: there are 40 quintillion black holes making up about 0.04% of the total cosmic energy budget, or about 10% of the total mass in stars. But how many are there as a function of mass, and how does that mass function evolve over time? With the next generation of gravitational wave observatories, these big questions of 2024 may be answered, with robust data, by the middle of the 21st century.