New black hole discovery proves it: ding, dong, the “mass gap” is dead
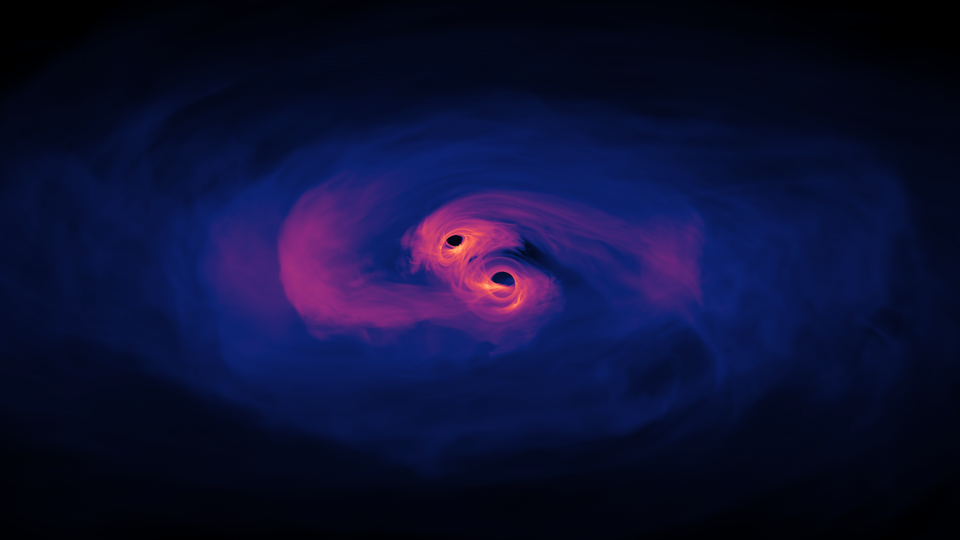
- Between the heaviest neutron stars and the lightest black holes, there was a “gap” where no objects were known.
- Since the dawn of gravitational wave astronomy, nearly 100 inspirals and mergers of stellar corpses have been seen.
- With the latest LIGO/Virgo data release, we now see there are no gaps at all; the only gap was in our ability to see them.
How massive can the most massive neutron star be, and how light can the lightest black hole be? For the entire history of astronomy up until 2015, our understanding of both of these phenomena was limited. While both neutron stars and black holes were thought to have formed by the same mechanism — the core-collapse of a massive star’s central region during a supernova event — observations only revealed low-mass neutron stars and black holes whose masses were significantly higher. While neutron stars seemed to top out at around twice the mass of the Sun, the least massive black holes didn’t appear until we got up to around five solar masses. This in-between region, puzzlingly, was known as the “mass gap.”
Starting in 2015 with the twin LIGO detectors, however, a fundamentally new type of astronomy was born: gravitational wave astronomy. By detecting the ripples in spacetime that emerged from the inspiral and merger of these very objects — black holes and neutron stars — we could infer the nature and masses of both the pre-merger and post-merger objects that resulted. Even after the first and second major data releases, this mass gap, perhaps puzzlingly, still persisted. But with the latest data release bringing us up to nearly 100 total gravitational wave events, we can now finally see what many had suspected all along: there is no mass gap, after all. There was only ever a gap in our observations. Here’s how we learned what’s truly out there in the Universe.
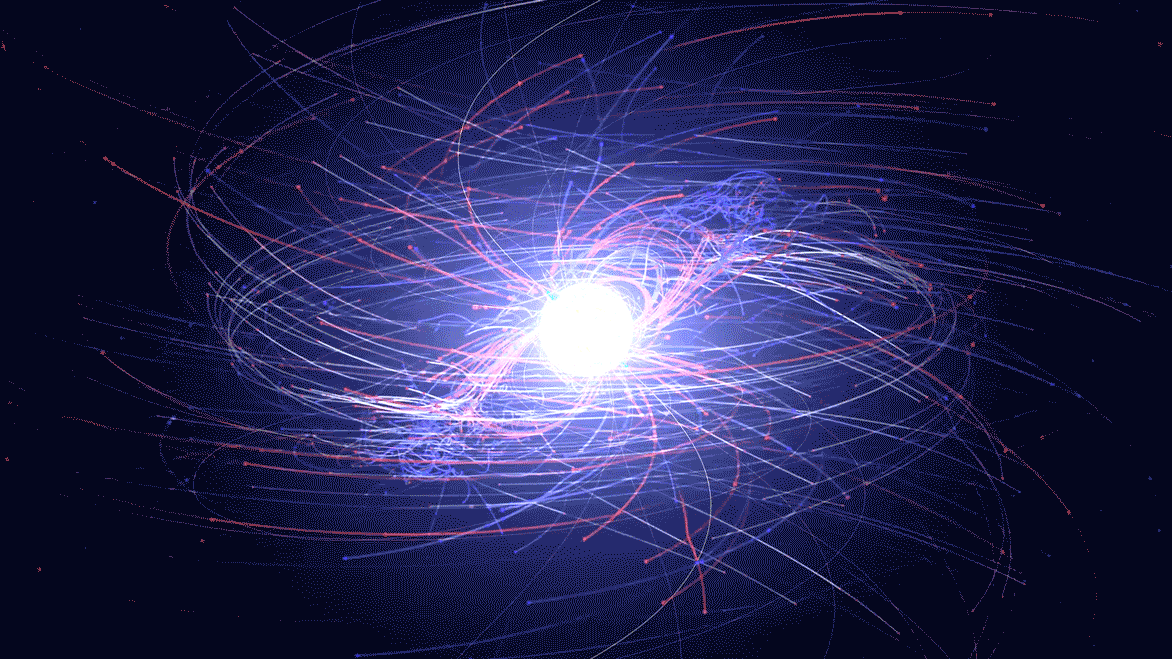
Before we ever saw our first gravitational wave, we already knew a fair bit about both neutron stars and black holes. Neutron stars were small, compact, rapidly rotating objects which served as sources of electromagnetic emissions, particularly at radio wavelengths. When a neutron star’s radio emissions passed across Earth’s line-of-sight, we would observe a brief radio pulse. If the neutron star rotates in such a fashion that its radio emissions crossed our line-of-sight once per rotation, we observed these pulses periodically: as a pulsar. Largely from observations of pulsars, both in isolation and as part of binary systems, we were able to find large numbers of pulsars up to about two solar masses. In 2019, the record was broken when a team led by Dr. Thankful Cromartie discovered a pulsar whose mass was 2.14 solar masses: the most massive neutron star directly observed.
On the other side of the equation, we had black holes, observable in two different classes. There were the stellar mass black holes, which we could detect when they were in binary systems from electromagnetic emissions emerging from various processes such as mass siphoning and accretion by the black hole. There were also supermassive black holes, largely observed at the centers of galaxies, detectable from their emissions and also from their accelerations of both the surrounding stars and gas.

Unfortunately, the black holes that were revealed by these methods were either extremely massive, like millions or billions of solar masses, or they fell into a relatively narrow range: about 5 to 20 solar masses. That was it. It led many to believe that there were potentially “gaps” in the masses of objects. One of these gaps was at the high end: above 20 solar masses. Another was at the low end: between about 2 and 5 solar masses. Part of the reason the prospect of LIGO, Virgo, and other gravitational wave observatories was so exciting is that, in principle, they’d be able to probe both of those ranges.
If there truly was a mass gap in either of those locations, and our gravitational wave detectors were as good as they were expected to be, they should have been sensitive to both of those populations. Lower-mass objects, as a part of binary systems, would be observable for relatively long periods of time, so that even though the signal amplitude is small, we can “build up” enough orbits to observe either neutron stars or low-mass black holes as they inspiral and merge, provided they’re close enough to us. Higher-mass objects, on the other hand, could be farther away, but only their finals very few orbits would likely be detectable. As a result, gravitational wave observatories, like LIGO, would have different distance ranges over which to be sensitive to these differing types of events.
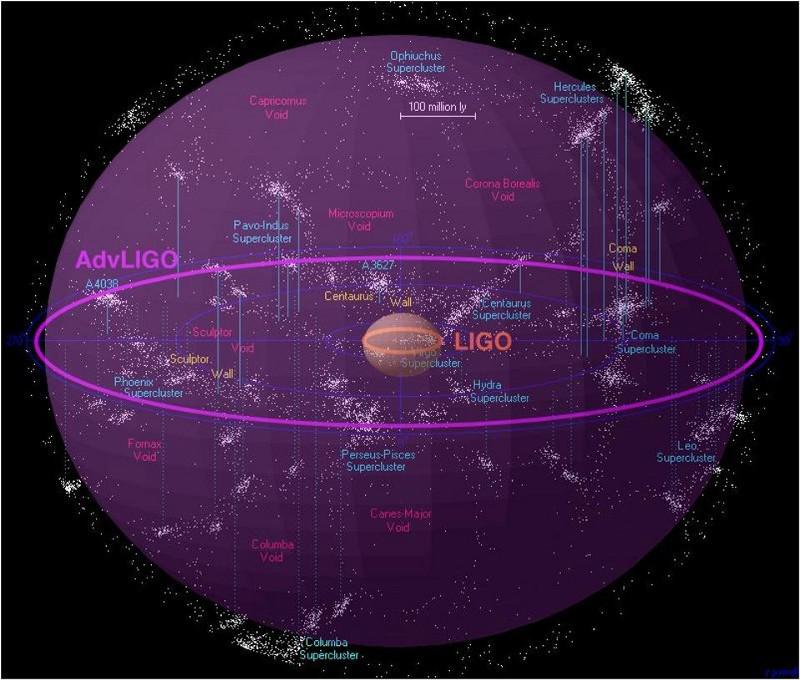
Remarkably, it was only days after the observatory first began taking data, back in September of 2015, when the first astrophysical signal appeared in our detectors. Immediately, this first event was unlike anything else we had ever seen. From over a billion light-years away, ripples in spacetime arrived, indicating the merger of two black holes that were each more massive than any of the stellar mass black holes we’d seen previously. Whereas the black holes that we had identified from their emitted X-rays from siphoning mass off of a companion topped out at 20 solar masses or so, this very first black hole-black hole merger revealed two black holes of 36 and 29 solar masses, respectively, merging into a 62 solar mass black hole.
The remaining three solar masses, meanwhile, were converted into energy via Einstein’s most famous equation: E = mc2, and it was that very radiation that enabled us to detect the merger that occurred so far away and so long ago. In one fell swoop, the first detection opened up the possibility that the “gap” above 20 solar masses wasn’t actually there, and was simply an artifact of what we were capable of detecting. With a new way of viewing the Universe, this population of more massive black holes was suddenly being revealed for the first time.
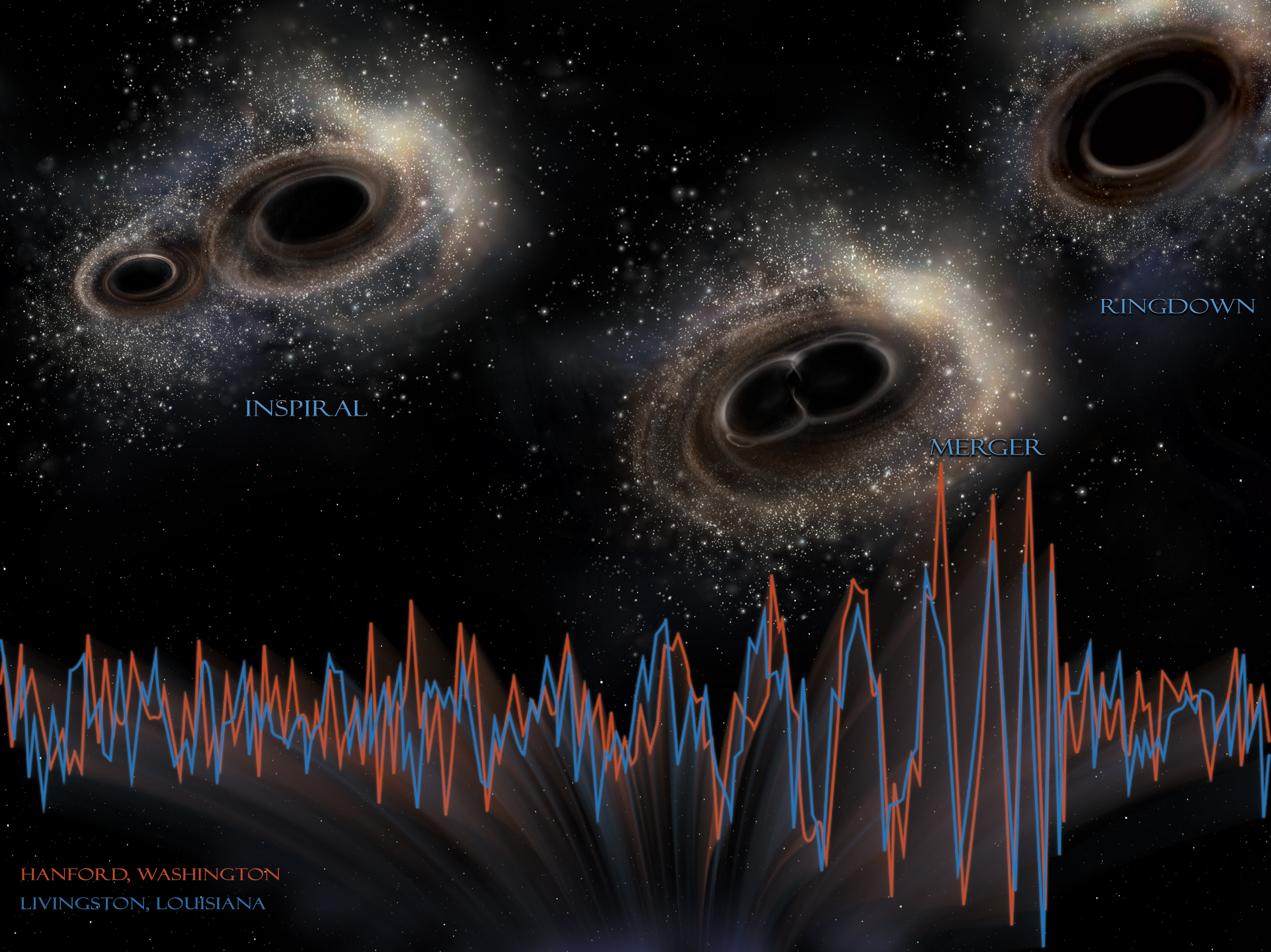
If you think about it, it makes sense that this population would be far more difficult to detect. The X-ray binaries that we had found — revealing the black holes we had found from electromagnetic emission, rather than gravitational waves — had two things going for them.
- They were all systems located extremely close by: only thousands of light-years away, almost exclusively in our own galaxy.
- They were all systems where a large, massive star was orbiting a black hole.
This information, on its own, explains why lower-mass black holes, of 20 solar masses and below, would be commonly seen by the X-ray emissions of their interactions with a companion, while higher-mass black holes wouldn’t be seen. When new stars form, the heavier you are in mass, the rarer you are and the shorter you live. When you form pairs of stars (i.e., binary systems), they tend to have comparable masses to one another. Therefore, if you’re restricted to sources within a single location, like the Milky Way galaxy or even our Local Group, the less likely you are to have a higher-mass X-ray binary in there, since you have less time where one member is a black hole and the other is still a star, and you simultaneously have fewer such objects at high masses.
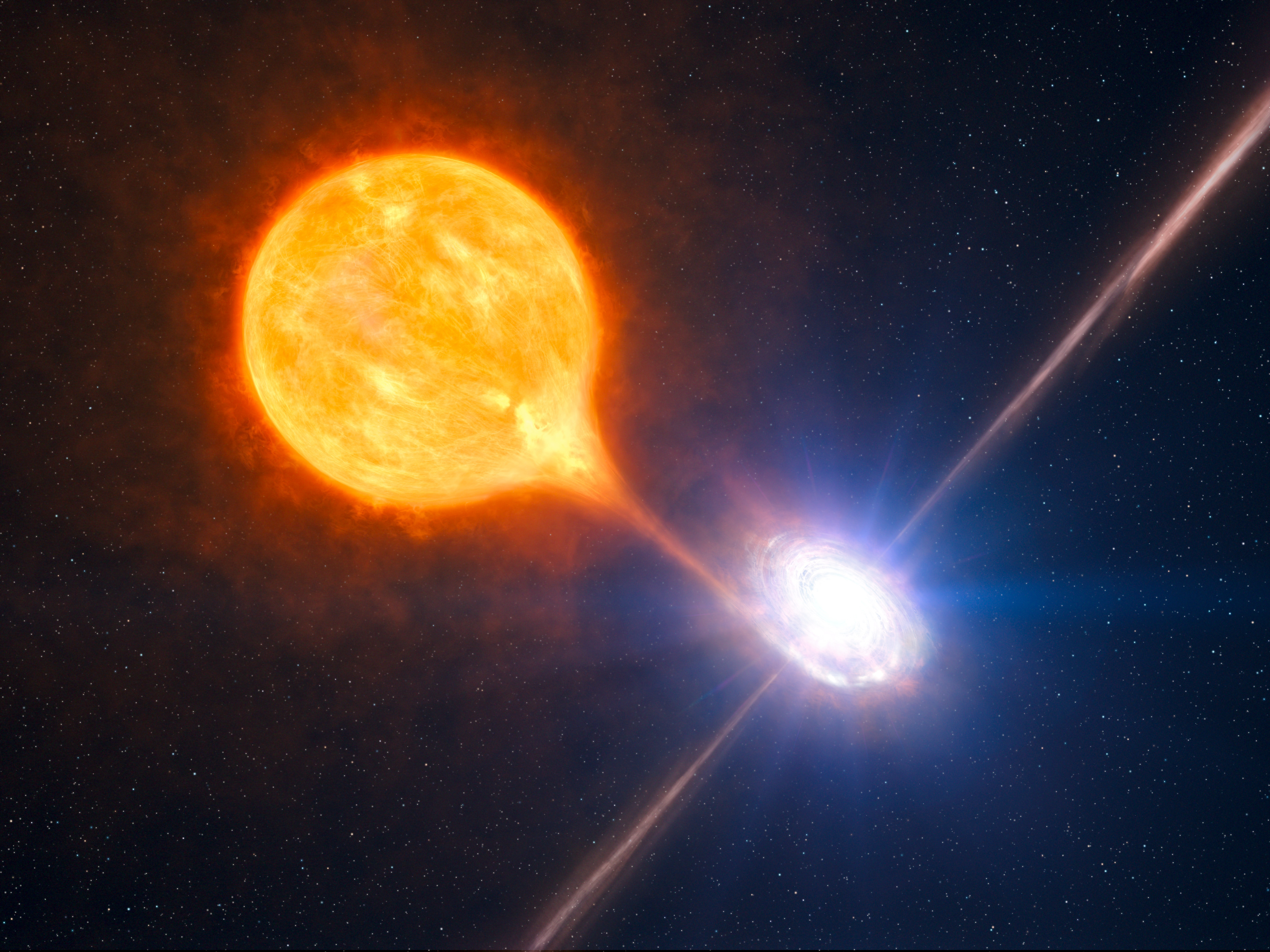
Gravitational wave detectors, meanwhile, can probe enormous volumes of space, and are actually more sensitive (i.e., can probe greater volumes) when it comes to detecting higher mass pairs. There isn’t the same time restriction for gravitational wave detectors, either, since the stellar corpses that form binary black holes will remain as binary black holes until they inspiral and merge. Remember: While electromagnetic signals, like light, have their flux fall off as one over the distance squared, gravitational waves are detected not through flux but through their strain amplitude, which falls off as simply one over the distance.
A greater-amplitude signal, generated by greater-mass black holes, can be seen significantly farther away than a lower-amplitude one, meaning that the LIGO (and Virgo) detectors are actually fantastic for probing the higher-mass regime of binary black holes, all the way up to the limits of LIGO’s frequency sensitivity. This corresponds to masses of right around 100 solar masses.
With nearly 100 total detections under our belt, we’ve seen that there’s a healthy population of black holes out there between about 20 and 100 solar masses, with no indication of a “gap” anywhere where we can observe, all the way up to the very top.
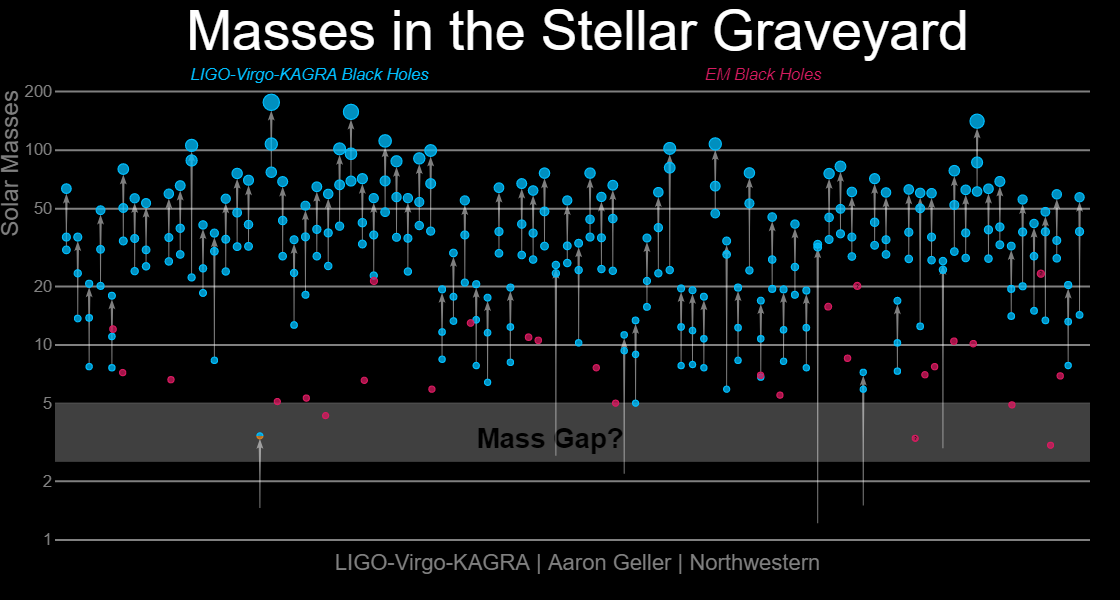
But what about on the other end: between 2-and-5 solar masses? That one was a bit trickier. Whereas even the first two data-taking runs of the LIGO scientific collaboration had revealed a copious number of black hole-black hole mergers of a wide variety of masses, there was only one event where anything fell into that “mass gap” range. That 2017 event, of a neutron star-neutron star merger just ~130 million light-years away, was one of the most educational events we had ever observed.
With the ripples in spacetime from that event arriving over the span of a few seconds, this was the first time a neutron star-neutron star merger had been seen in gravitational waves. Less than 2 seconds after the gravitational wave signal ceased, a gamma-ray burst event was spotted. Over the next few weeks, dozens of space-based and ground-based observatories all turned towards the now-identified location, the galaxy NGC 4993, to follow-up with observations across variety of electromagnetic wavelengths. This kilonova event, in many ways, was a Rosetta Stone towards uncovering not only the nature of neutron star-neutron star mergers, but also the nature of the mass gap.
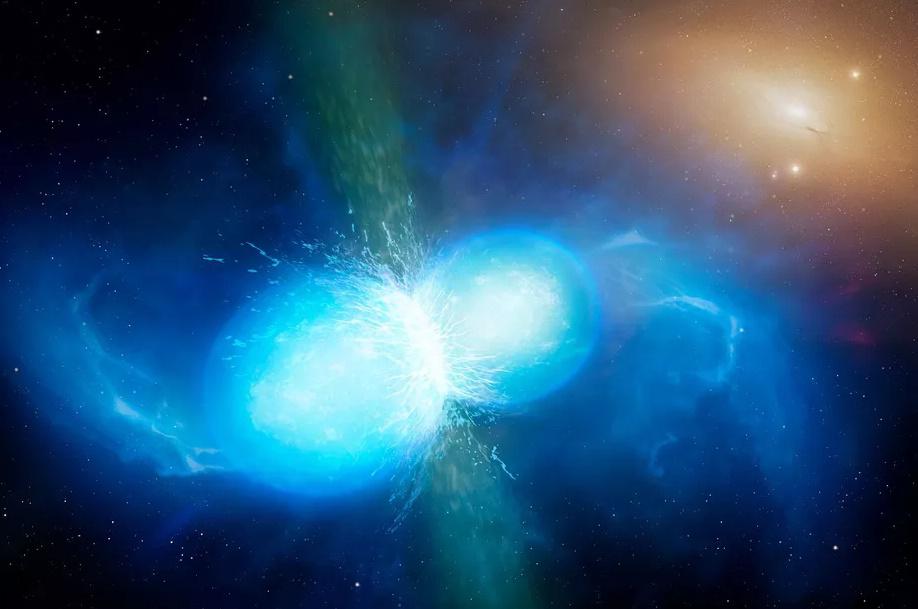
In theory, just as there’s a limit to how massive a white dwarf star can get before the atoms in their core collapse, triggering a type Ia supernova, there’s a similar limit to the masses of neutron stars. At some point, the degeneracy pressure between the subatomic particles in the neutron star’s core will be insufficient to prevent further collapse into a black hole, and once that critical threshold is crossed, you can remain a neutron star no longer.
It isn’t just the mass of the object that this depends on, but its spin as well. In theory, a non-spinning neutron star might collapse to a black hole at around 2.5 solar masses, while one spinning at the physically allowable limit might remain a neutron star all the way up to 2.7 or 2.8 solar masses. And, in one final piece to the puzzle, an asymmetric object — one not in hydrostatic equilibrium — will gravitationally radiate energy away until it reaches an equilibrium state in a sort of “ringdown” effect.
So, what did we conclude from the data we gathered about that August 17, 2017 event? That two neutron stars, one of about the mass of the Sun and one quite a bit more massive, merged together, producing an object in the range of 2.7 to 2.8 solar masses. Initially, that object formed a neutron star, but in just a few hundred milliseconds, it collapsed to a black hole. Our first object in the mass gap had just been found, and wow, was it ever an informative doozy.

In the subsequent years, a second neutron star-neutron star merger was seen, but this one had more massive progenitors and the final product was somewhere between 3 to 4 solar masses. With no electromagnetic counterpart, we conclude that it became a black hole directly. Still, even after that, scientists wondered where all of these 2.5-to-5 solar mass black holes were, as we didn’t generally see progenitor black holes involved in mergers of that mass. Even after these discoveries, there was ongoing discussion about the existence of a mass gap, and whether there was a dearth of black holes in this mass range for some reason.
With the latest and greatest data release from the LIGO and Virgo collaborations, where fully three of the latest 35 new events fall into this “mass gap” range, we can finally put that idea to bed. There may be a slight difference in the rates of black hole mergers in the below-5-solar mass range compared to the above-5-solar mass range, but what’s observed is consistent with the expected rates based on the present sensitivity of our detectors. With the evidence for a mass gap having evaporated with better data and greater statistics, there’s no longer any reason to suspect that there’s an absence of stellar remnants in that range in any remarkable way at all.
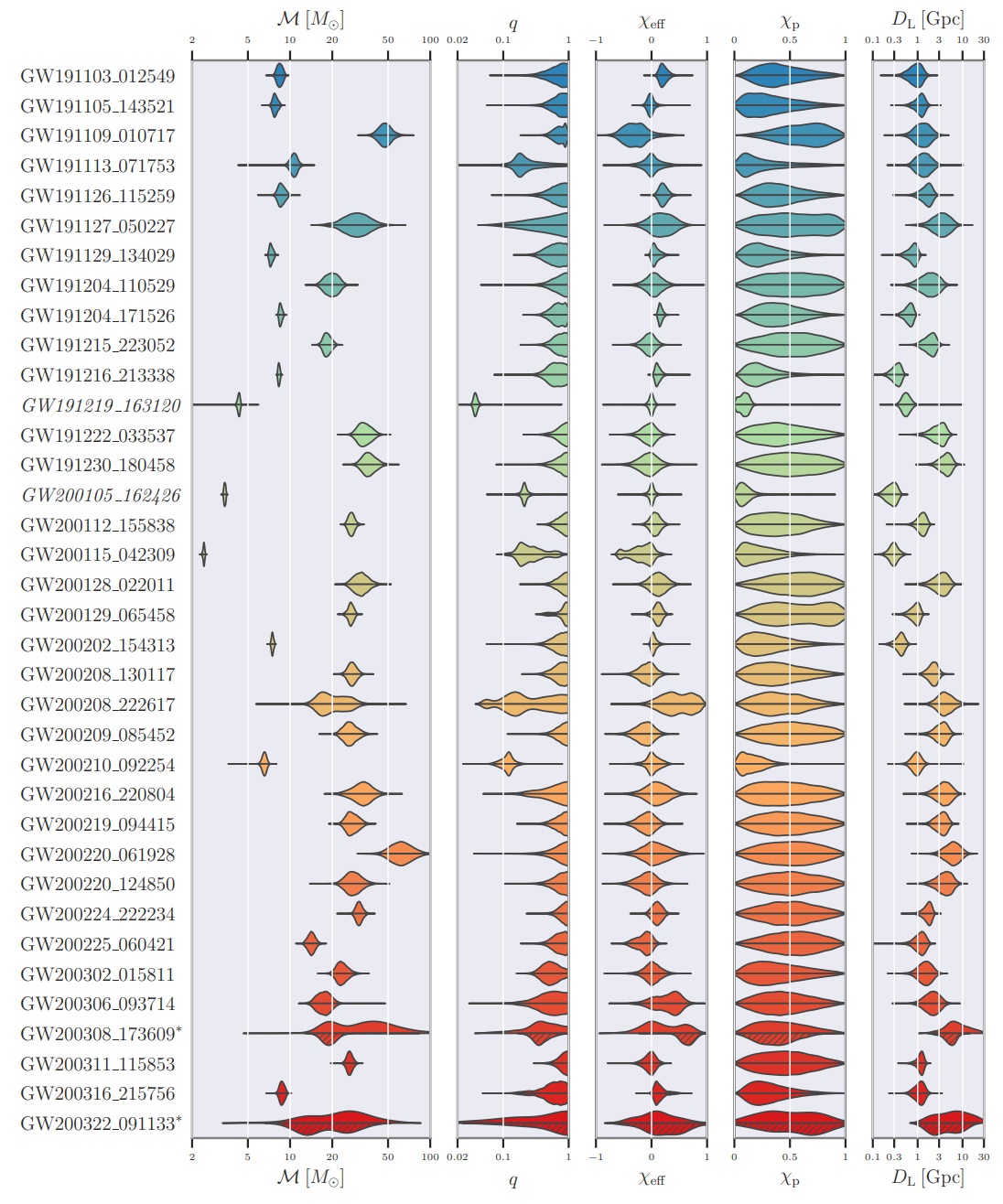
As little as four years ago, there was no substantial evidence for black holes or neutron stars in the 2-to-5 solar mass range, leading many to question whether there might be a “mass gap” for some reason: where these ubiquitous stellar remnants were somehow forbidden. Maybe, it was reasonable to conclude, that dying massive stars either made a neutron star, capping out at about ~2 solar masses, or a black hole, which didn’t begin until ~5 solar masses, and that the only objects in between would be exceedingly rare: the product of a merger between two neutron stars, for instance.
That is definitively no longer the case.
With the latest findings from gravitational wave astronomy, it’s become clear that neutron stars and black holes in the 2-to-5 solar mass range are seen with precisely the frequency that our technology allows us to observe them. Not only that, but their observed abundances appear to be in agreement with expectations from stars and stellar evolution. What was once a curious absence has now been shown, with better data and improved statistics, to have been there all along. It’s a simultaneous showcase of both the great and self-correcting power of science, while also cautioning us against drawing too-strong conclusions from insufficient, premature data. Science isn’t always fast, but if you do it properly and patiently, it’s the only way to guarantee you’ll get it right in the end.