What was it like when no stars yet existed?
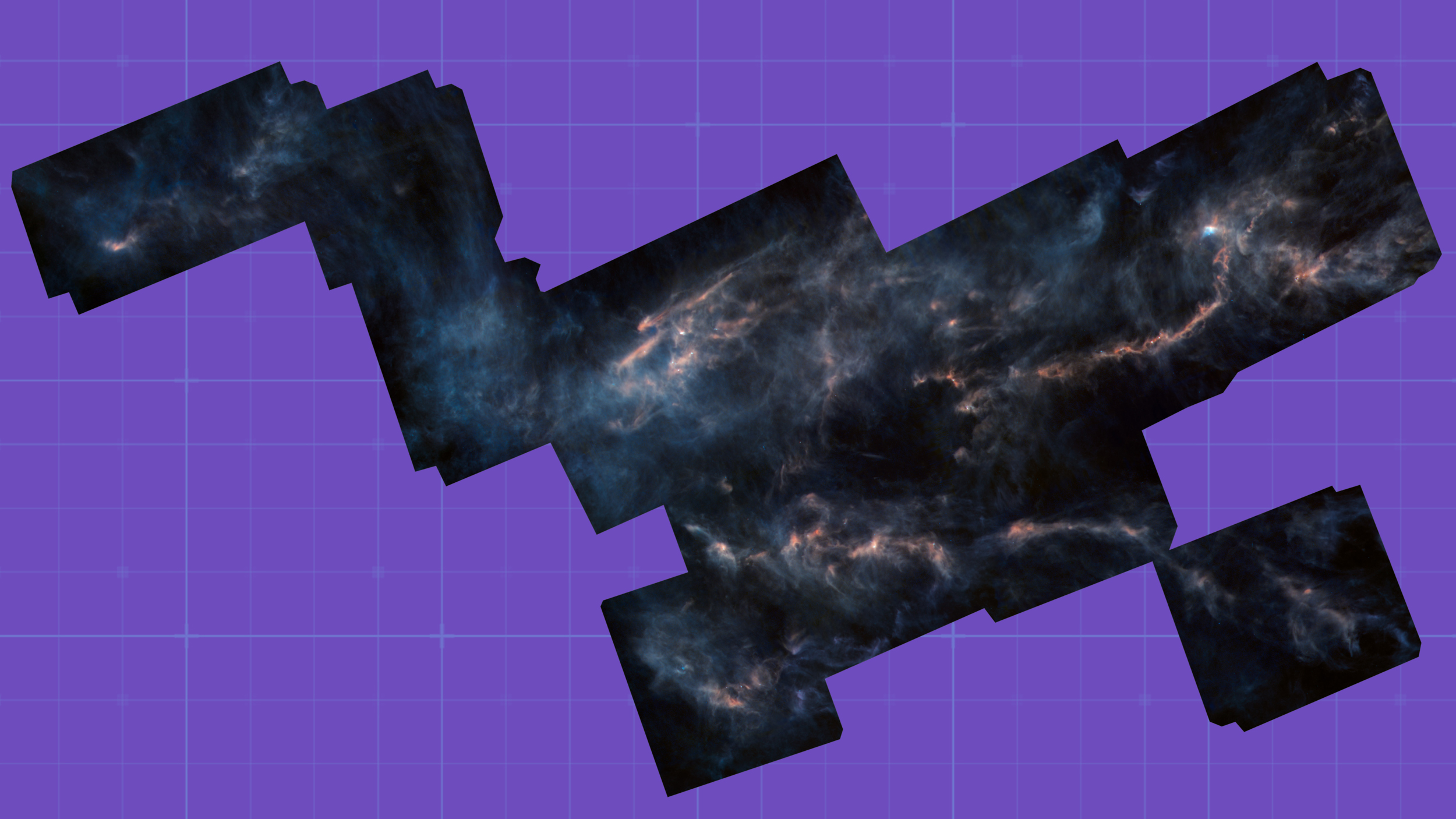
- After the hot Big Bang, it took minutes for atomic nuclei to form and then hundreds of thousands of years to make neutral atoms, but the first stars wouldn’t form until nearly 100 million years had passed.
- Those in-between times, after neutral atoms form but before the first stars were created, represents a unique, darkness-filled time in the Universe’s history: the cosmic dark ages.
- What was the Universe like during that time, when no stars yet existed but neutral atoms were everywhere? It’s a fascinating epoch that science is only beginning to explore.
When it comes to our cosmic history, it’s incredible to realize how impactful the earliest moments were in creating the conditions that would allow for our very existence many billions of years later. The earliest stages we can say anything meaningful about actually occurred even prior to the start of the hot Big Bang. Cosmic inflation took place and then ended, seeding the Universe with quantum fluctuations and then giving rise to the hot Big Bang. The Universe cooled and expanded from its hottest, densest stages to produce more matter than antimatter, then stable protons and neutrons, then atomic nuclei, and eventually even neutral atoms, all amidst a background sea of radiation and neutrinos.
You might think that once neutral atoms form, the next step would be driven by gravitation: the formation of stars. But the timescales required to form them are immense compared to everything that came before. By the time just half-a-million years have passed, the Universe is dominated by matter, the radiation sea is cool enough that atoms cannot become ionized, and gravitation gets to work in earnest. Even with those ingredients, it will still take somewhere between 50 and 100 million years for even the very first star in the Universe to form. For all the time in between, the Universe experiences the darkest part of the era known as the cosmic dark ages. Here’s what it was like back then.

The formation of neutral atoms isn’t simply important for setting into place the building blocks of all the complex chemical structures that can arise from molecules, ions, and any combination of atoms bound together. It’s also very important for “freeing” the photons, or particles of light, left over from the hot Big Bang. When neutral atoms first formed, that marks the time when photons stopped scattering off of free electrons, since free electrons are only present when your atoms are ionized in the form of a plasma. Once all the neutral atoms form, radiation then simply travels in a straight line; with nothing to scatter off of, it simply moves at the speed of light.
This light, in terms of the number of photons that are present, vastly outnumbers the number of atoms in the Universe. The light now appears to come uniformly from all directions and in all locations. At the start of the cosmic dark ages, the temperature of this photon bath starts off at 2970.8 K, which would appear yellow-orange in color at that moment. Some regions are slightly hotter than others, reaching temperatures of up to 2971.0 K, while others are slightly cooler, at around 2970.6 K. Those minuscule differences might not seem like much, but it’s the most important factor in how our Universe will evolve and grow from this point forward.
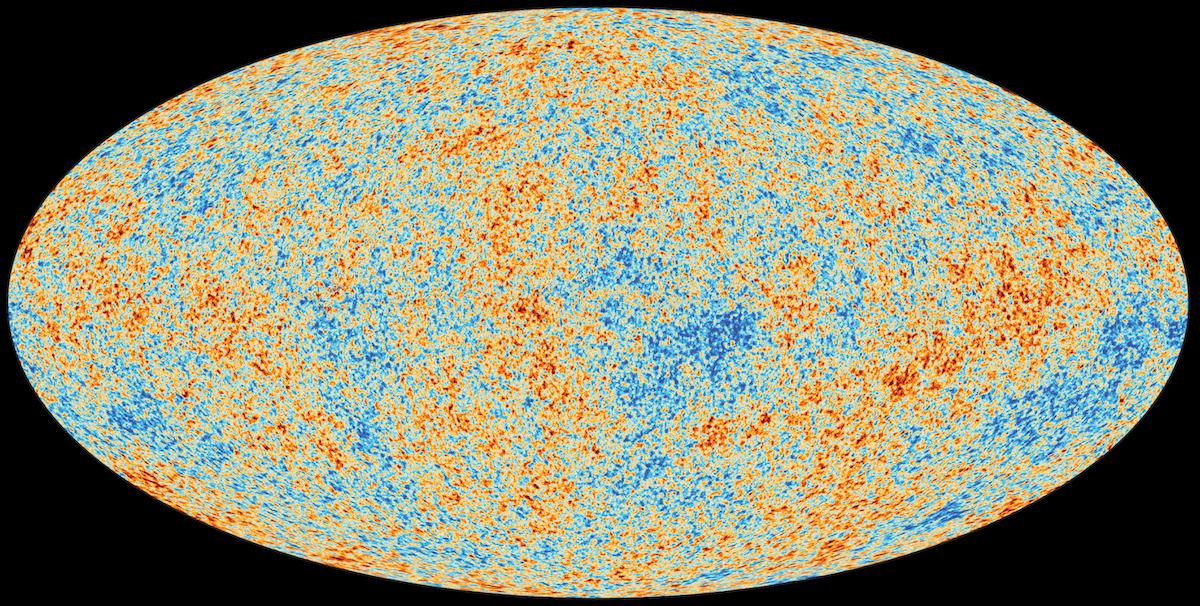
Why do these tiny temperature differences matter? Because in every region of space, all of those photons, or particles of light, actually both have the same amount of inherent energy and also have that energy distributed in the same fashion among all the photons present. The radiation, intrinsically, actually carries the same amount of energy in the hot regions as it does in the cold regions to start with, but the environment in which that radiation lives varies slightly from location to location. Some regions have exactly the average density that the overall Universe does, but other regions have slightly more (or less) matter than average.
The underdense regions, since they have less matter in them, have less gravity in them. When a photon travels out of that region, it has a smaller gravitational potential to fight against, meaning it loses less energy due to gravitational redshift, creating a photon temperature that appears hotter than average.
On the other hand, the overdense regions have more matter in them, and therefore, they have more gravity to fight against. As the photons climb out, they lose more energy than average, and therefore the photons emerging from those regions become colder, or less energetic, overall.
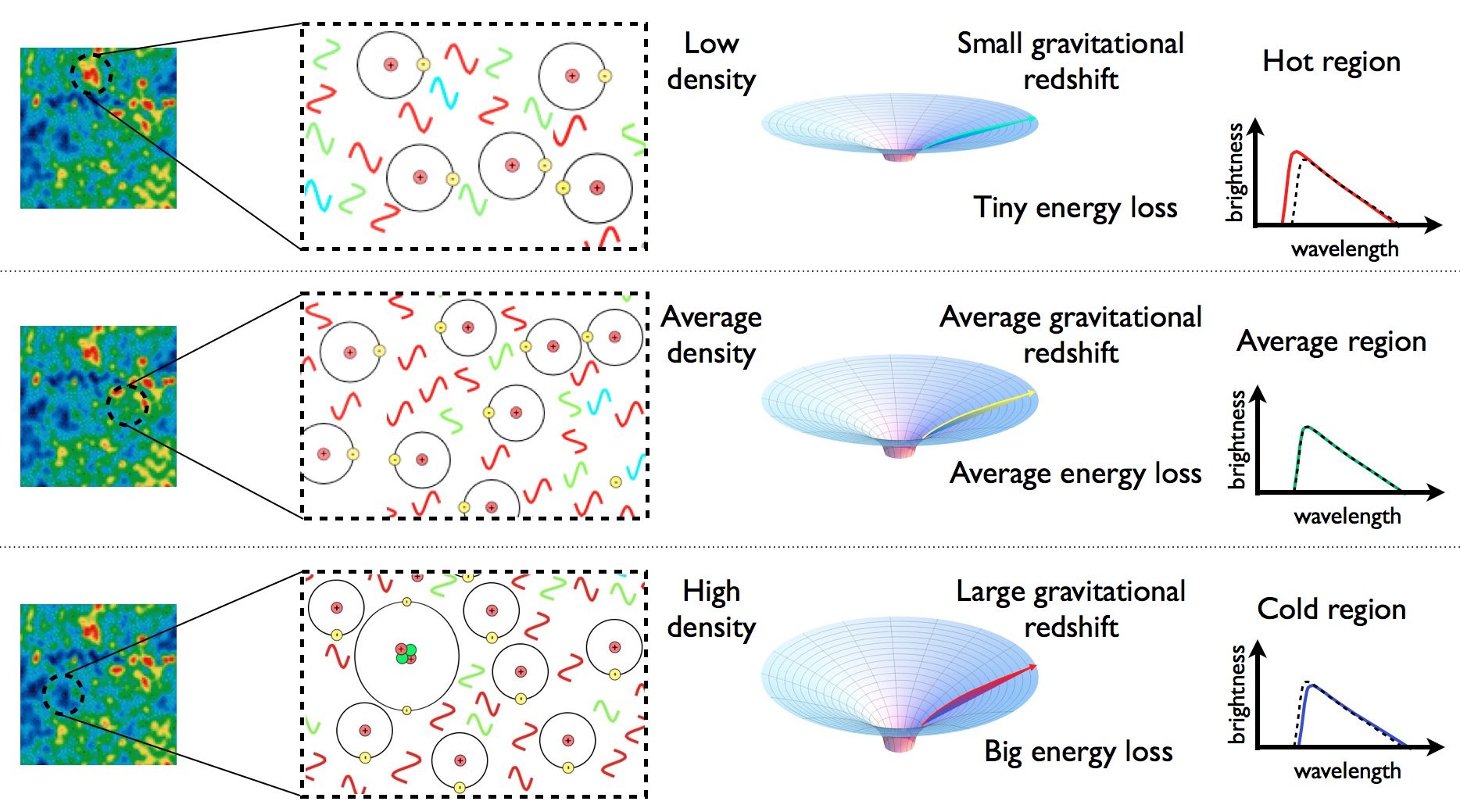
If you have a Universe with regions that are:
- of average density,
- of greater-than-average density,
- and of below-average density,
you might think that all that’s left to do is for these overdense regions to clump together and attract more and more matter, as gravity dictates, until we form stars. That’s part of the story, but it turns out that’s not the only factor at play. The photons, as a part of the Universe, have a little more to add to the story before they simply fade into the cosmic background.
The way gravity works is similar to how you might intuit it in your mind: all the masses attract one another, and wherever you have the most mass, it preferentially pulls in all the other mass around it. Even in the expanding Universe, these overdense regions attract mass from any nearby region that’s less dense, particularly from the underdense regions, which can only tenuously hold onto their matter, at best.

What this teaches us is that gravity, in this sense, is a runaway force. In terms of drawing mass into a region, there are going to be “winners” and “losers” in this Universe, and the regions that have the largest head starts are the ones that wind up with the most matter in the end. As more and more matter gets attracted into a region, the more successful gravity then becomes at bringing even more additional matter into it.
However, this isn’t the full story. As true as this is, matter and gravity aren’t the only major presences in the Universe in action at this time. There’s also radiation, in the form of this leftover background of photons. And while matter — both dark matter and atomic matter — gravitationally attracts massive particles, it draws all forms of energy, including radiation, into the most overdense regions.
When this occurs, we have to remember that radiation, unlike matter, has a significant amount of pressure inherent to it. Our Sun, for example, has 300,000 times the mass of Earth, but is less dense than our own planet, and the reason for that is the tremendous outward pressure exerted by photons inside of it. The same type of pressure that holds a star like our Sun up against gravitational collapse also can hold up these collapsing gas clouds before any stars have formed, slowing the rate at which they grow. Even in a matter-dominated Universe, so long as radiation is still important, the matter overdensities can only grow very slowly.
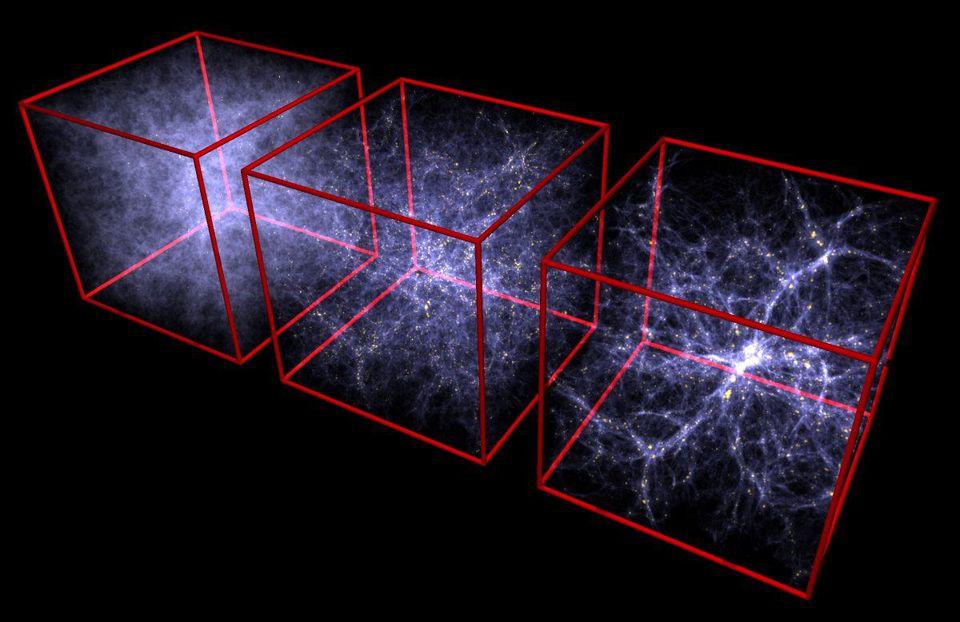
For millions of years, the rate of growth of all these types of cosmic structure, driven by gravity, is severely limited. Radiation pressure simply will not allow the matter density to grow any faster than at a certain specific rate. Because we have millions of years that need to pass while the matter density grows and grows, even in the densest regions, another process begins to take place: something inherent to the most common type of atom in the Universe: hydrogen. Ever since we’ve formed neutral atoms out of (mostly) protons and electrons, there’s a new form of light that gets emitted: the light from the spin-flip transition within hydrogen atoms.
During these dark ages, 92% of the atoms in the Universe are simple hydrogen. Hydrogen atoms are made out of one proton and one electron, and both protons and electrons possess an intrinsic spin to them: either +½ or -½. There is a slight difference in the overall energy between a system where the proton and electron have the same spin (either +½, +½ or -½, -½), making it slightly higher-in-energy than where they have opposite spins (either +½, -½ or -½, +½). On timescales of around 10 million years, the configurations where they have the same spin will spontaneously flip, emitting a photon of a specific wavelength, 21 cm, when that happens.

Although the radiation present in the Universe is primarily driven by the leftover photons from the Big Bang, which today is observable as the cosmic microwave background radiation, there’s now another signal that gets added atop that one: this weak, tiny signal from the 21 cm emission line. If 92% of the atoms in the Universe are these simple hydrogen atoms, and there’s a 50/50 chance of protons and electrons having initially aligned or anti-aligned spins when they make these atoms, then that means there should be a 21 cm emission signal emerging from approximately 46% (by number) of all the atoms in the Universe.
Every hydrogen atom that spontaneously forms in a state where the protons and electrons are aligned will emit light in this fashion, and will do so every time new hydrogen atoms are made, including from previously neutral hydrogen atoms that were then ionized again. Although we have yet to detect the 21 cm emission signal emerging from the very first atoms formed in the Universe, it’s a signal that we know how to predict, and that a sufficiently advanced radio telescope should be able to detect if enough observing time is dedicated to uncovering it.
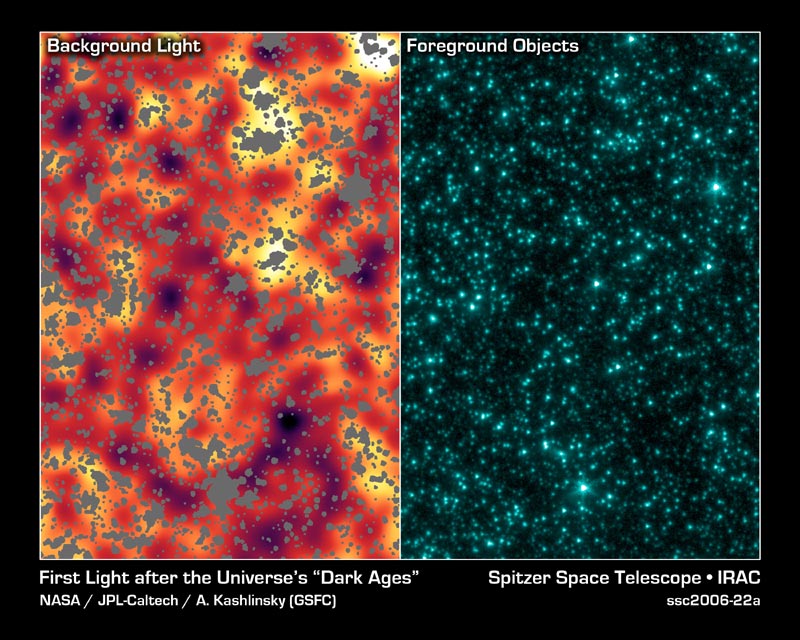
But there are other processes happening simultaneously that will be even more important for the cosmic story that’s only just beginning to unfold. While it’s true that the normal matter in our Universe — the stuff that makes up all the stars, planets, and all of the chemical reactions that will ever occur — is arguably, from a human-centric point of view, the most important component of our reality, there’s a lot more than just this normal matter within the Universe we know. The first step toward the creation of those entities will be the formation of the first stars, but we’ve still got a long way to go to get there from the moment we form neutral atoms.
We need help in order to get there: the help of photons redshifting, the help of gravitation pulling matter into the overdense clumps, and the help of time for both of these effects to build up to have substantial impacts. Over the first 3 million years after neutral atoms form, the temperature cools from ~3000 K to 800 K, taking the radiation from yellow-orange to orange to red colors, after which it finally cools enough to become invisible to human eyes. The falling radiation pressure allows matter clumps to grow, but only to about four times the magnitude that they were when the CMB was emitted.

By the time the Universe is between 15 and 20 million years old, it’s cooled to around the temperatures we experience here on Earth: empty space is about room temperature. The matter clumps that were just a little bit denser than average (maybe 1-part-in-30,000) have grown in amplitude significantly, and are now approximately 10-15 parts in 30,000 denser than the cosmic average. The densest clumps have started to grow somewhat more rapidly, and may be up to 60-to-90 parts in 30,000 denser than average: about 0.2% or 0.3% denser than a typical region of space.
It’s very important to keep track of how dense these overdense regions get, because there’s a critical density that matter can reach where the simplest explanation for how these clumps grow no longer applies. Up until that point, in what’s known as the linear regime of structure formation, the overdense regions grow as though they follow a straightforward law: when the Universe is half the temperature, the matter clumps grow to double their original overdensity. Once you pass a certain, critical threshold, the clumps start growing much more quickly, in what we call a nonlinear fashion.
That critical transition happens once a region becomes about 68% denser than average. After that point, runaway collapse becomes inevitable.
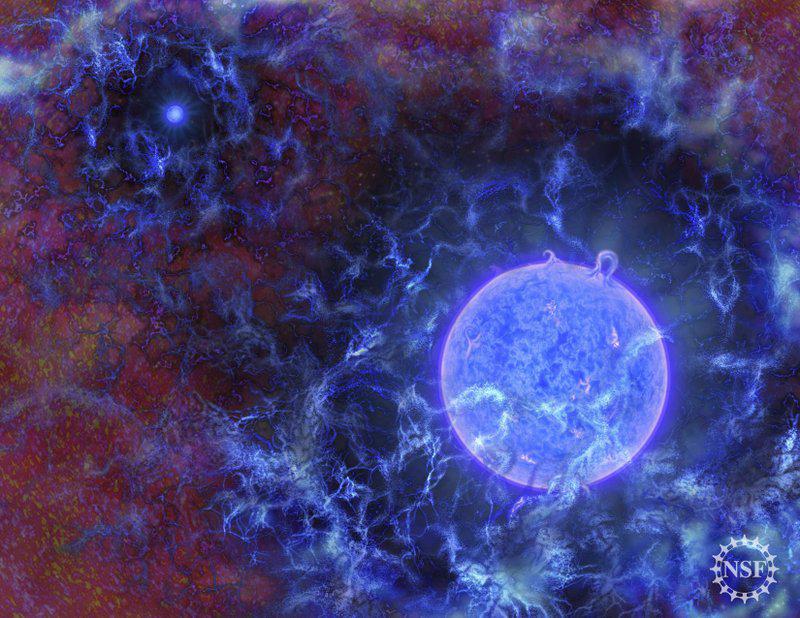
So when does this first happen? At some point when the Universe achieves about 50 million years of age (perhaps a little bit more), the densest clumps have now transitioned into this post-critical phase, and start contracting and drawing additional matter in at an extremely accelerated rate. This will rapidly lead to the formation of the first stars in the absolute densest regions of space, but most of the rest of the Universe will continue only growing slowly, requiring more time for the matter clumps to grow to densities where star formation is possible.
The first big waves of star formation, on large cosmic scales, won’t begin until the Universe is around 200-250 million years old. However, within the very densest regions, the matter within them collapses down to large densities in just 50-to-100 million years. At some point, due to cooling, the very first star — defined by the first hydrogen-into-helium chain reaction via proton-proton fusion — will occur. In a Universe filled with dark matter and normal matter, the Universe must cool to somewhere around 100 K before the first true star can actually form.

Conditions, just before the first stars formed, were significantly different to how they are today. Space wasn’t transparent to light, but rather was filled with neutral, light-blocking atoms. The leftover glow from the Big Bang, today’s CMB, was about 30-to-50 times hotter than it is now back then. And these conditions, within the expanding Universe, occurred so long ago that even with its powerful infrared capabilities, even JWST cannot observe them. Even though many hope that JWST will enable us to directly view those first major waves of star formation, the very first stars of all should remain obscure even to its unprecedentedly powerful eyes.
It takes under half a million years to take all the normal matter in the Universe and to complete the formation of neutral atoms, but 100-to-200 times as long before that neutral matter can collapse down enough to form even the very first star in the Universe. Until that happens, the only light to see will be the leftover glow from the Big Bang, which falls to low enough energies to make it invisible to what human eyes would see after just 3 million years. For the next 47-to-97 million years to follow, the entire Universe is truly dark.
But once the first star ignites, “let there be light” will finally, once again, become a part of our cosmic history.