What was it like when the very first stars died?
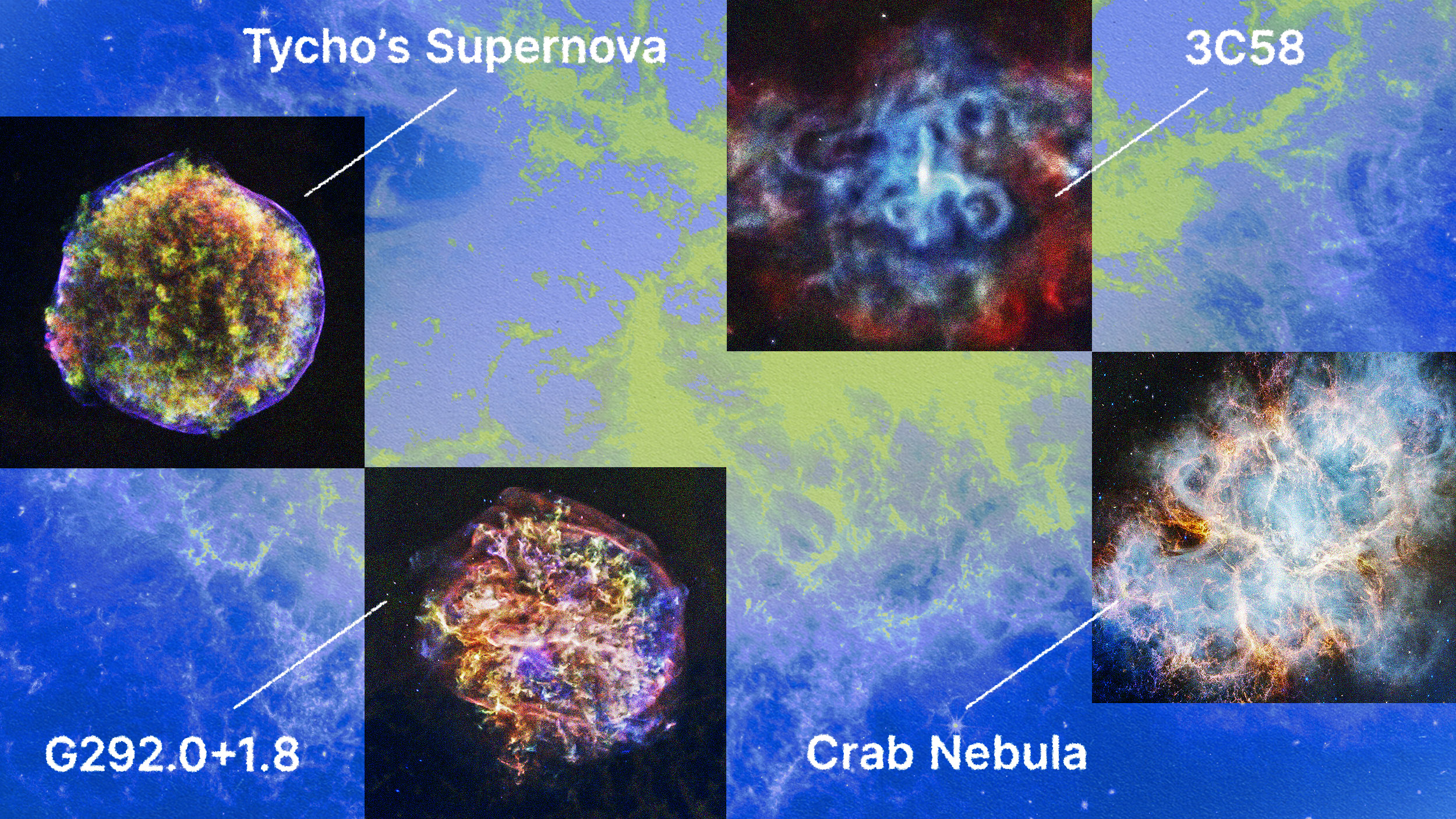
- Once the very first stars formed, with such high masses, the shortest-lived ones would die after only one or two million years: an incredibly short stellar lifetime.
- Although they were initially formed only out of hydrogen and helium, a great many of these stars would be responsible for crafting heavy elements inside of them: enriching the Universe when they die.
- This cosmic enrichment is one of the most important parts of the story that led to us: the creation of the atoms of the periodic table. Here’s how the first stars dying helped make it all happen.
The cosmic story that gave rise to us is a story rife with creation and destruction. At the start of the hot Big Bang, energetic particles, antiparticles, and quanta of radiation were created. Fractions-of-a-second later, most of the particle-antiparticle pairs had annihilated away. Protons and neutrons formed within the first second, and then over the subsequent minutes, atomic nuclei fused together, creating the first elements. Over the next several hundred thousand years, neutral atoms finally formed, and gravitation pulled matter together into clumps. Eventually, some of the largest clumps gravitationally collapsed, creating the first stars.
But these stars, made up of the pristine material forged in the hot Big Bang, would not remain the only luminous objects in the Universe for very long. As these stars were overwhelmingly massive, 25 times the typical mass of stars created during modern times, they burned through their fuel rapidly, causing them to evolve through their life cycles extremely quickly. The more massive a star is, the shorter its lifespan, meaning that these very first stars didn’t live for long at all. The death of the first stars was absolutely necessary to give rise to the Universe as we know it today. Here’s the cosmic story you haven’t heard.

In order to form stars, the gas you’re going to make it out of needs to collapse. But gravitationally collapsing means you have to radiate energy away; the very act of collapsing is one of energy transfer, where gravitational potential energy gets turned into kinetic energy, and where the kinetic energy of matter (i.e., the energy of motion) causes that material to heat up. Today, heavy elements are the best and most efficient energy-radiators that exist, which means that clouds of gas can collapse efficiently, and form all sorts of stars, from the rare ones that come in at hundreds of solar masses down to very small, faint ones at the low-mass end: right at the lower limits of what defines a star.
Early on, however, there were no heavy elements, since those only arise from stars in some way. The first stars, therefore, can only be made out of large clumps of matter that have enough mass to overcome the effects that come from being unable to efficiently radiate your heat away. This is the primary reason behind why the first stars are very large and massive: 10 solar masses on average, with many stars reaching up to hundreds of solar masses and likely with a few that crest the 1000 solar mass threshold, which is unheard of at modern times.

This leads to a seemingly paradoxical statement that I simply call the Blade Runner conundrum. In Blade Runner, one of the main characters is told, “The flame that burns twice as bright lives but half as long,” and while that might be true for flames, the situation is even worse for stars. It’s true that the more massive a star is, the brighter it burns, and the shorter it lives, but “twice as bright” for “half as long” is a gross understatement. While a star like our Sun might live around 10-12 billion years before reaching the end of the fuel in its core, these early stars, with hundreds or even thousands of times the mass of our Sun, are expected to have a lifetime of ~0.01% our Sun’s: just 1-2 million years, before they meet their demise.
As the cores of these stars fuse hydrogen into helium at an incredibly rapid pace, they give off thousands-to-millions (or more) the luminosity of our Sun continuously. For a star ten times the mass of our Sun, that process might last only around 10 million years before running out of hydrogen fuel, whereas more massive stars have even shorter lifetimes. At that point:
- the core contracts and heats up, fusing helium into carbon,
- when it runs out of helium, it heats up and fuses carbon into neon and oxygen,
- and then burns oxygen up to form magnesium, silicon, and sulfur,
- eventually reaching iron, nickel, and cobalt,
- and then ending in a spectacular supernova explosion.
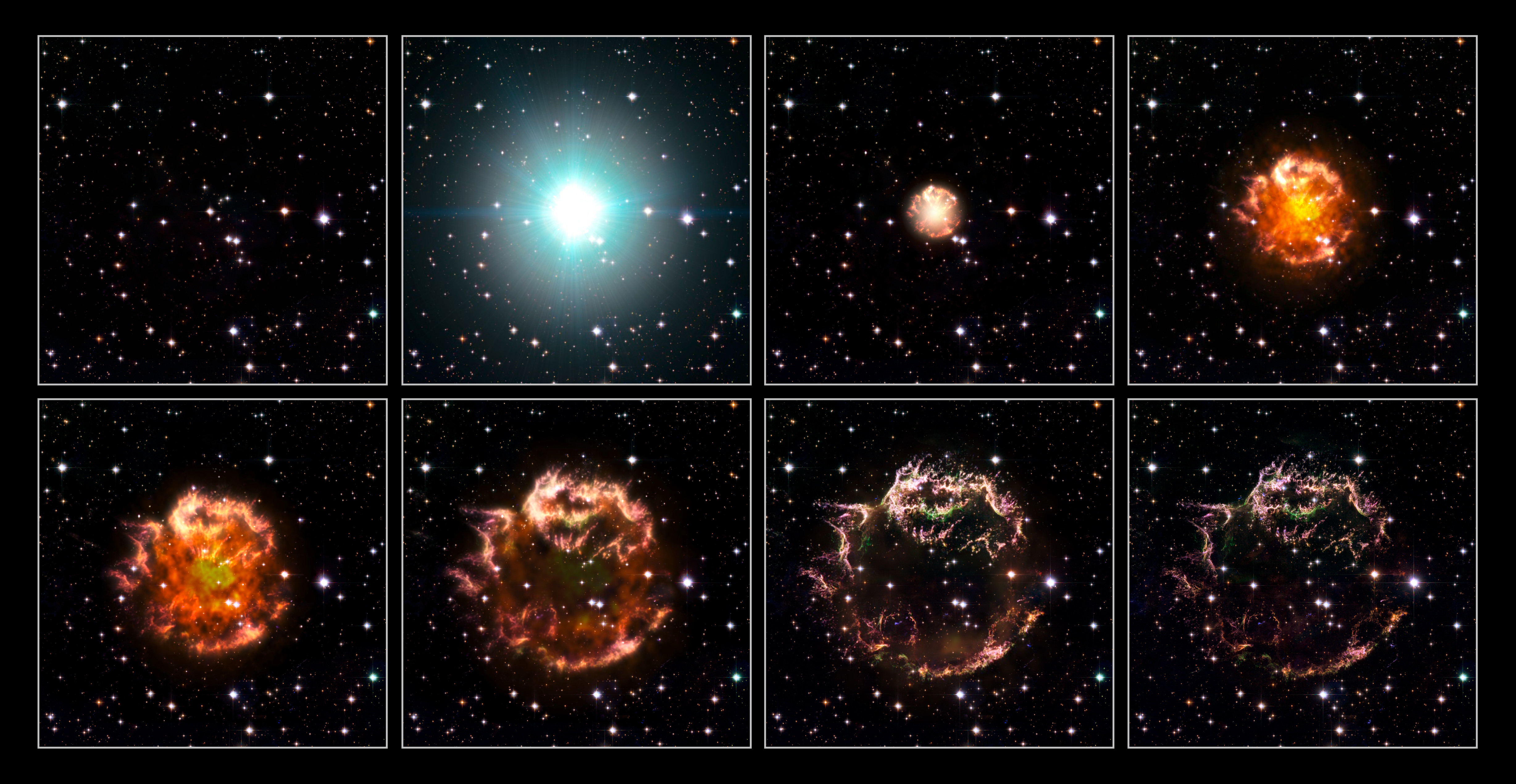
The cycle of nuclear fusion in massive stars creates a large amount of heavy elements in the periodic table, building elements up to around iron (element 26), and then a supernova occurs. Those elements then experience a rapid bombardment by neutrons as they get blasted off back into the interstellar medium at the moment of the supernova’s detonation, where they typically rise up the periodic table to reach zirconium (element 40), with heavier elements capable of being formed out of those now-enriched, heavier-than-ever-before elements.
What that supernova leaves behind, which is the former core of the progenitor star, is typically a neutron star: a collapsed mass that’s greater than our Sun, but no bigger than perhaps a dozen miles from end-to-end. The most massive stars that go supernova are likely to form black holes rather than neutron stars, but the majority of stars that undergo a core-collapse supernova will be between 8-and-40 solar masses, and are much more likely to leave a neutron star behind.
That’s not even the end of the story, as within these dense, early environments, neutron star-neutron star collisions should be relatively common, giving rise to kilonova events.

When these neutron star collisions occur, they give rise to either a larger neutron star or a black hole with about 95% of their combined mass, which you might anticipate. But these neutron star collisions also result in runaway, explosive reactions, causing the emission of gravitational waves, neutrinos, electromagnetic radiation of all types, and the expulsion of large quantities of heavy nuclei. These nuclei come in both stable and unstable varieties, and typically climb all the way up the periodic table, producing the majority of the heaviest elements that are ever created in the Universe.
While merging neutron stars are responsible for a large fraction of the elements niobium, molybdenum, tin, tellurium, barium, some of the lanthanides, as well as a little bit of mercury and lead, they produce the overwhelming majority of all the other elements heavier than zirconium, including elements that are far heavier than even uranium and plutonium. In combination with supernovae, neutron star-neutron star mergers help give rise to the full suite of elements that make up the periodic table, including the absolute heaviest elements that naturally occur.

The “average” star, among the first stars, might be around 10 times the Sun’s mass, and might have just 0.1% of the Sun’s lifetime, dying in a supernova after around 10 million years or so. But as is frequently the case with “averages,” a great number of stars will be of above-average mass, and those stars will live for even shorter periods of time. There are stars hundreds or even a thousand times as massive as our Sun that get created here, and they burn through their fuel even more quickly. Shining as bright as millions or even tens of millions of Suns, each one has a unique fate.
In general, there are three mass-dependent possibilities for what will happen to such a star.
One is just a higher-mass analogue of what you’d expect from the earlier supernovae: a massive supernova that leaves only a black hole, rather than a neutron star, behind. The core of a supernova collapses, and in most cases, that’s going to lead to a neutron star. But there’s a limit, somewhere between 250% and 300% the mass of the Sun, to what a neutron star can achieve before it collapses under its own gravity. When it crosses that threshold, the neutron star collapses all the way into a black hole: the second most common fate for the first stars.
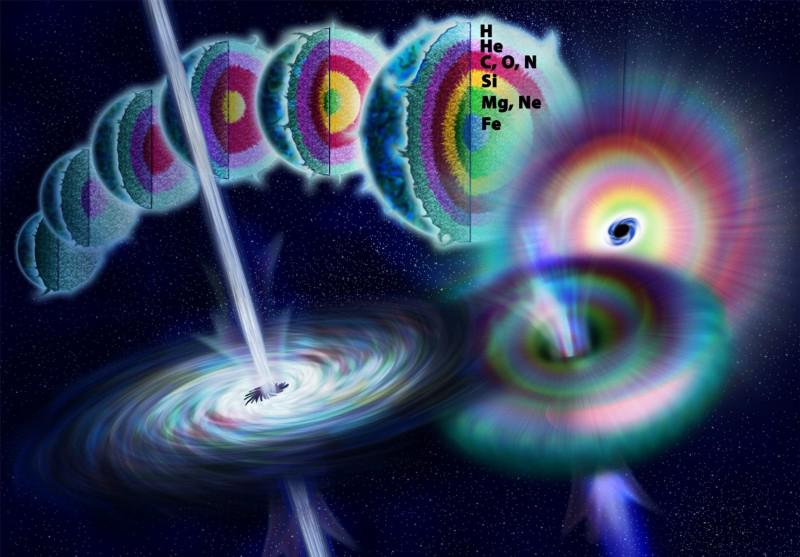
At even higher masses, however, the temperatures inside the star reach such great levels that a special process begins to take place. There’s enough free energy that, for the photons flying around inside the star’s core, there’s the possibility that they can spontaneously form particle-antiparticle pairs. Two photons can spontaneously transform, under these conditions, into an electron and positron, if energies are high enough.
This carries with it some new physics: whereas the radiation pressure from the photons was what held the star up against gravitational collapse, the loss of photons means a loss of pressure, and the star begins to collapse further. As it does, the temperature goes up, making it more likely that photons will convert into electron-positron pairs. This becomes a runaway process, and the star’s core collapses entirely.
This process is then known as a pair-instability supernova, or, if you prefer colorful language, a hypernova explosion. These are extremely rare in the modern Universe, but the first stars should have had many instances of this type of cataclysm. The less massive pair-instability supernovae will lead to a black hole at the core, while blowing off their outer layers, while the more massive ones will destroy the star entirely, giving rise to a much more severely-enriched portion of the interstellar medium in the vicinity where they occurred.

It’s theorized that stars of different masses will reach the pair-instability threshold at different times in their life cycles, making the elements they expel and enrich the Universe with a variable that is not yet well-understood. In general, though, we have a pretty good theory of how, assuming that no major mass-transfer events occur to either add mass to the star or siphon mass off of it, a star will evolve, live, and die based solely on two factors: its initial mass and the fraction of heavy elements, or metallicity, that it’s born with.
The biggest uncertainties in this calculation, perhaps unsurprisingly, are to be found at the extreme low-metallicity end: for the stars made out of the most pristine material. Because all of the stars we observe at present are no longer pristine, but have been thoroughly enriched with material that’s passed through multiple generations of stars in the past before forming the stars that are now present today, it’s very difficult to find a star with less than a certain fraction (about 0.1%) of the heavy elements found in our Sun. Although we think that pair instability supernovae are common, as are normal supernovae that create black holes or neutron stars, those aren’t the only options.

Finally, stars of either severely extreme masses or that undergo just the right set of processes could experience a fate that doesn’t result in a cataclysmic explosion at all: no supernova of any type. Instead, these massive stars could directly collapse into a black hole. There doesn’t need to be a runaway fusion reaction for this to occur; there might not be an explosion or any ejecta; the mass could, all at once, simply overcome the radiation coming from its central region, leading to gravitational collapse. And once an event horizon forms, collapse down to a black hole is all but inevitable.
Although this might seem like a science-fiction scenario, we actually have some remarkable evidence that this occurs in nature, not only for “pristine” stars but even at late times. All across the Universe, there have been stars that were imaged at one or more points in the past, but when we look back today, at where they once were, they appear to simply have vanished. There’s no radiation in any wavelength of light, nor is there any remnant that we can see despite all the ways we know of to look for them. It appears, somehow, as though these massive stars have simply directly collapsed: likely into a black hole.

It’s theorized that the process of direct collapse, either from the most massive stars or from clouds of gas that never passed through a stellar phase, is responsible for the origin of the seeds of the supermassive black holes that occupy the centers of galaxies today. It may be the deaths of the most massive stars, which create black holes hundreds or thousands of times the mass of the Sun, that contribute, but it could also be clumps of matter that reached tens or hundreds of thousands of times the Sun’s mass that directly collapsed to create these seed black holes, which could then grow to the enormous masses they’re observed to have at late times.
Over time, mergers and gravitational growth will lead to the most massive black holes known in the Universe, black holes that are millions or even billions of times the mass of the Sun by today. It took perhaps 50-to-100 million years to form the very first stars in the Universe with many pristine stars still forming several hundred million years after the Big Bang. However, once you form stars, it takes only another million or two years after that for the most massive among them to die, creating black holes and spreading heavy, processed elements across the interstellar medium. As time goes on, the Universe, at long last, now contains heavy elements, and begins to resemble the Universe we know and inhabit today.