10 unbelievable but true facts about NASA’s James Webb Space Telescope
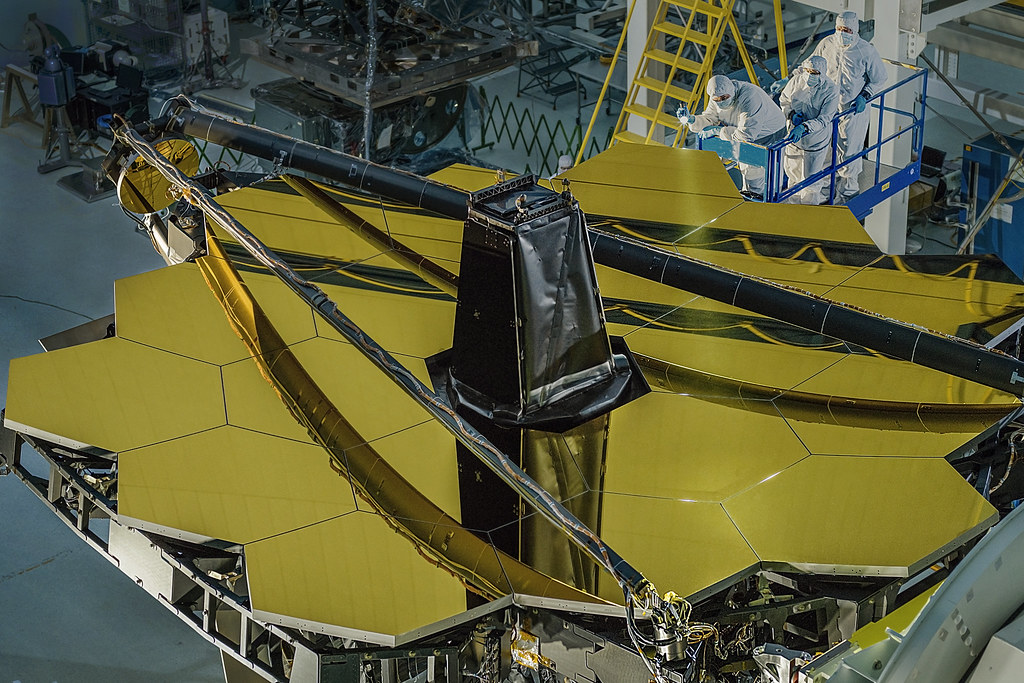
- On December 25, 2021, barring an unforeseen complication, the James Webb Space Telescope will launch from French Guyana.
- While astronomers hold their collective breaths, waiting for every necessary step to go right before science operations begin, we all can collectively appreciate what a marvel the telescope actually is.
- Here are 10 facts — trivia to some, the end result of a career of hard work for others — for everyone to enjoy.
The most delayed telescope in history is about to experience not merely a moment of truth, but a series of them over the coming few months. First, the telescope must survive its December 25 launch, which must point it precisely on course to the L2 Lagrange point. Then, it must successfully separate from the launch vehicle and then deploy its solar panels almost immediately. After that, the tower assembly, the sunshield, and the primary and secondary mirrors must all successfully deploy: steps involving hundreds of single-point-of-failure mechanisms. A series of thruster firings must also take place, eventually leading to Webb arriving at its destination: in orbit around the L2 Lagrange point.
If — and only if — all of these steps succeed, then NASA’s James Webb Space Telescope will begin taking data as never before, exploring the Universe with unprecedented power and an unrivaled series of instruments and capabilities. There are a series of discoveries we’re practically guaranteed to make once science operations begin, as well as the potential for discovering whatever resides out there amidst the vast ocean of the unknown cosmos.
And yet, despite all of that, it’s also worth appreciating some of the amazing and novel engineering that’s gone into the design and execution of this telescope. Without further ado, here are 10 incredible and hard-to-believe facts about NASA’s latest and greatest observatory: the James Webb Space Telescope.

1.) The James Webb Space Telescope is actually lighter than its predecessor, the Hubble Space Telescope. This one is a real shocker to most people. Under most circumstances, if you want to build a bigger version of something, it’s going to be heavier and more massive. For comparison:
- Hubble was 2.4 meters in diameter, with a solid primary mirror, and a collecting area of 4.0 square meters.
- James Webb is 6.5 meters in diameter, made out of 18 different mirror segments, with a collecting area of 25.37 square meters.
And yet, if we were to put them both on a scale here on Earth, we’d find that Webb has a mass of ~6,500 kg, or a weight of 14,300 pounds. When Hubble was launched, for comparison, it had a mass of ~11,100 kg and a weight of 24,500 pounds; with its upgraded instruments, it now has a mass of ~12,200 kg and a weight of 27,000 pounds. This is a tremendous feat of engineering, as practically every component on James Webb, where applicable, is lighter than its Hubble analog.
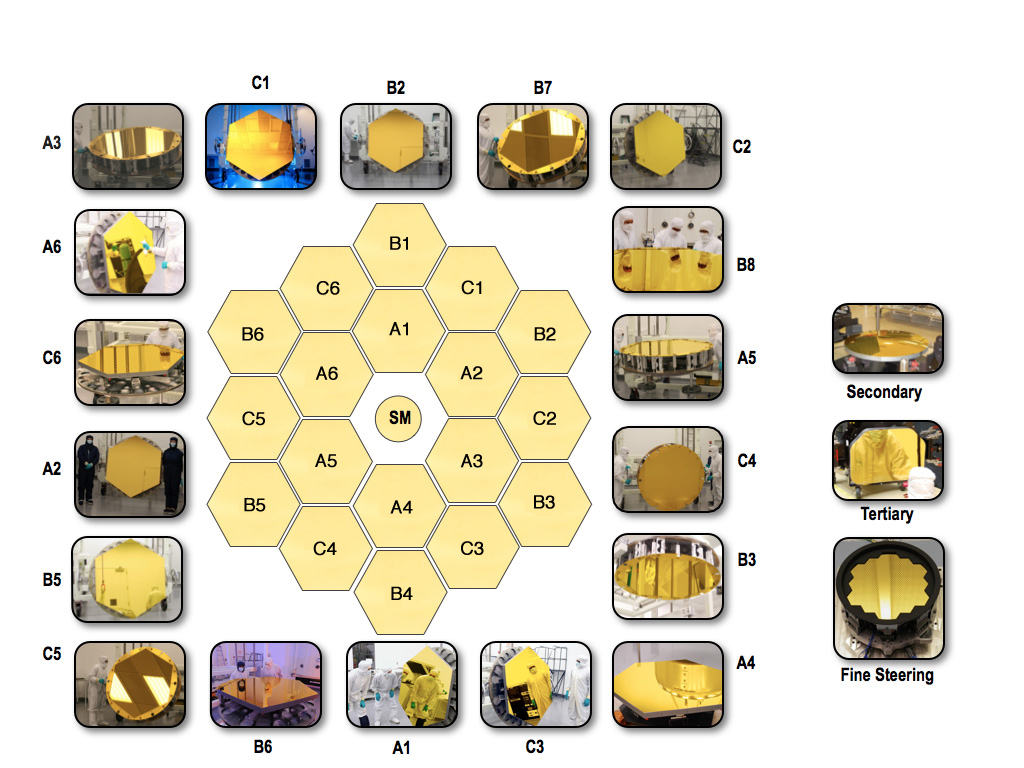
2.) James Webb’s mirrors are the lightest large telescope mirrors of all-time. Each of the 18 primary mirror segments, when it’s first manufactured, is in the shape of a curved disk, and possesses a mass of 250 kg (551 pounds). By time they’re completed, however, that mass has been reduced to a mere 21 kg (44 pounds), or a 92% reduction in weight.
The way this is accomplished is fascinating. First, the mirrors are cut into their hexagonal shape, which offers a slight reduction in mass. But then — and here’s where it gets brilliant — practically all of the mass on the “back side” of the mirror is machined away. What remains has been tested to ensure that it will:
- retain its precise shape even under the stresses of launch
- not break under vibrations and tension, despite its brittle nature
- survive the expected number and speed of micrometeoroid impacts
- be sensitive to the needed changes in shape that will be adjusted by the actuators attached to the back
All in all, these 18 mirrors will form a single mirror-like plane to an accuracy of 18 to 20 nanometers: the best of all-time, all with the lightest such mirrors ever manufactured.
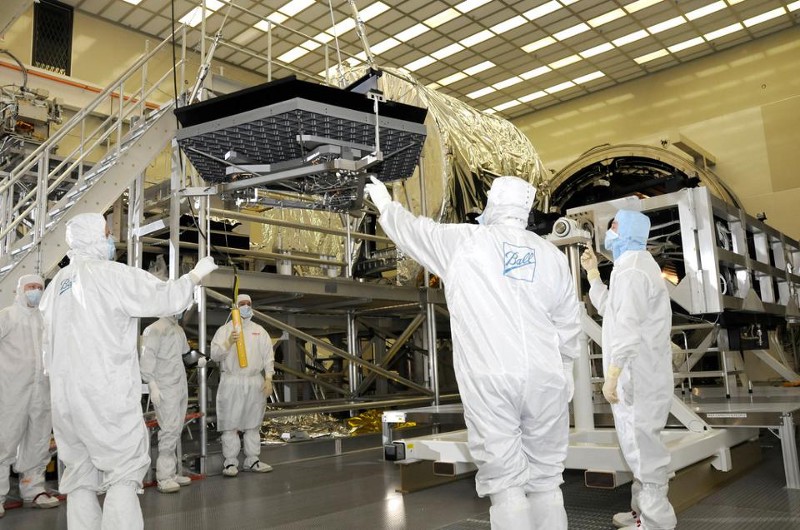
3.) Although they appear gold, James Webb’s mirrors are actually made out of beryllium. Yes, there’s a gold coating applied to each of the mirrors, but it would have been catastrophic to manufacture the mirrors entirely out of gold. No, not because of the very high density, nor because of gold’s malleability, both of which are properties it definitely possesses. The big problem would be thermal expansion.
Even at very low temperatures, gold expands and contracts substantially with small temperature changes, which is a dealbreaker for the material of choice for Webb’s mirrors. However, beryllium shines on this front. By cooling down beryllium to cryogenic temperatures and polishing it there, you ensure that there will be room-temperature imperfections, but that those imperfections will disappear when those mirrors are cooled again to operating temperatures.
Only once the beryllium is manufactured and machined to its final shape is the gold coating then applied.
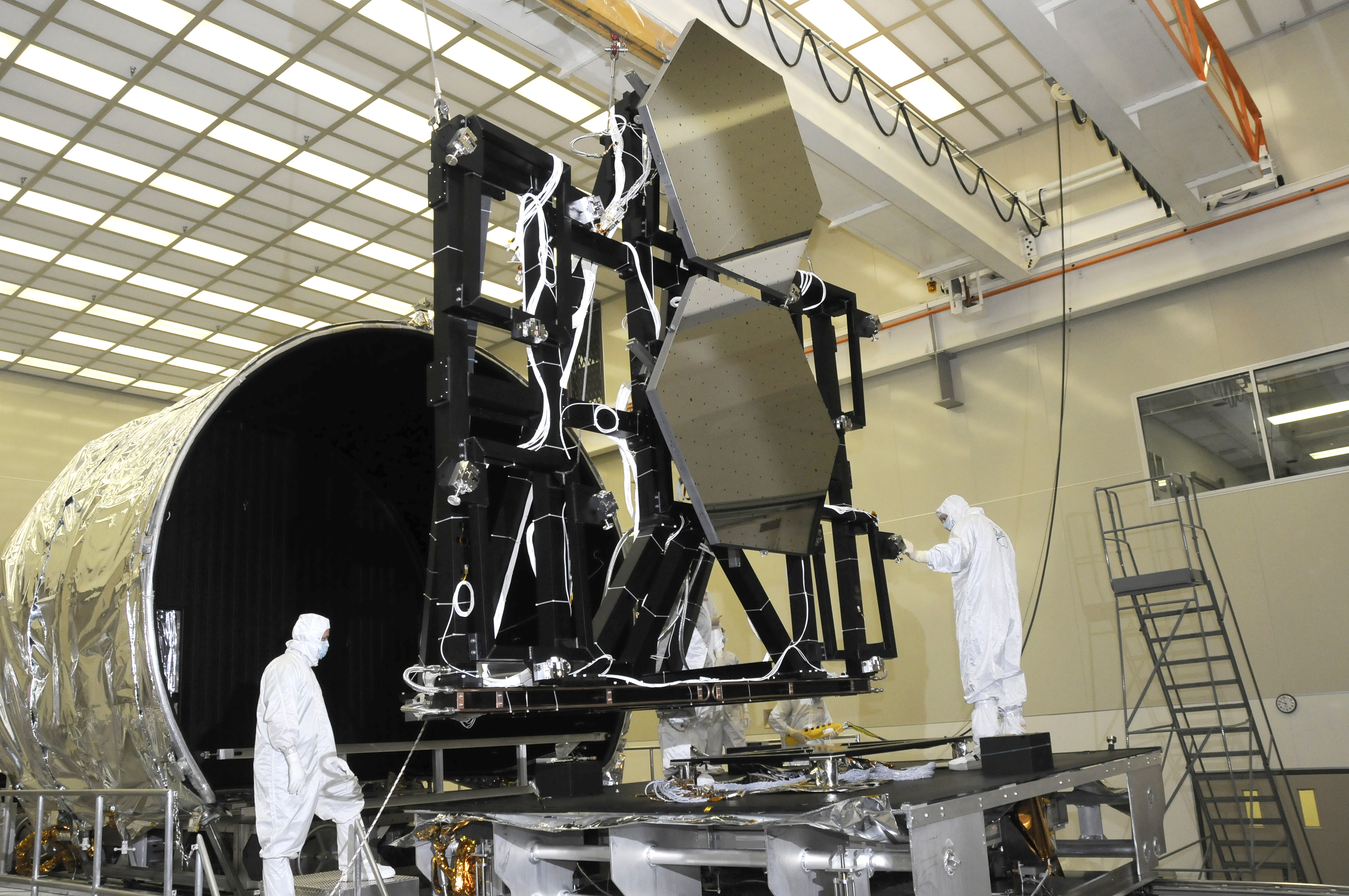
4.) The total amount of gold in the James Webb Space Telescope’s mirrors is only 48 grams: less than 2 ounces. Each one of James Webb’s 18 mirrors needs to be outstanding at reflecting the type of light it’s designed to observe: infrared light. The amount of gold applied needs to be just right; apply too little and you won’t cover the mirror entirely, but apply too much and you’ll start to experience expansion, contraction, and deformation when the temperatures change.
The process by which the gold coating is applied is known as vacuum vapor deposition. By placing the “blank” mirrors inside a vacuum chamber, where all the air is evacuated, you then inject a small amount of gold vapor inside. Areas that don’t need to be coated, like the back side of the mirror, are masked off, so that only the smooth, polished surface winds up coated with gold. This process continues until the gold reaches the desired thickness of only ~100 nanometers, or about ~600 gold atoms thick.
All told, there’s only 48 grams of gold in the James Webb Space Telescope’s mirrors, while the dull back sides get struts, actuators, and flexors attached to them.
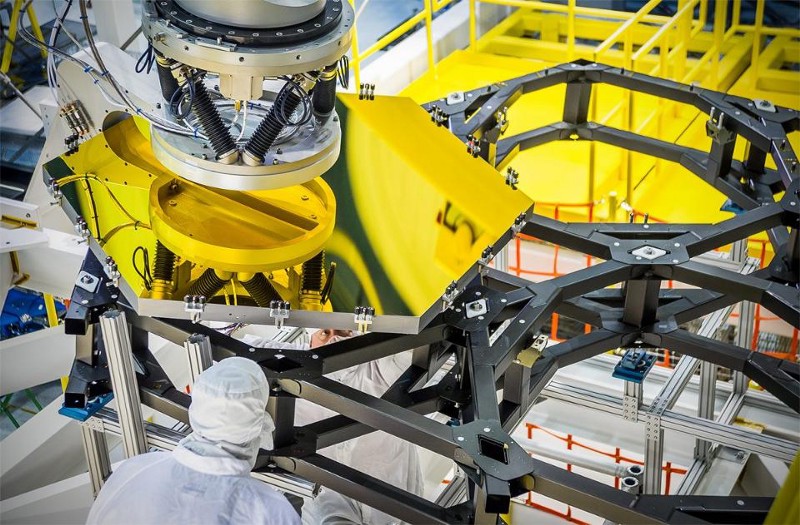
5.) The gold itself will not directly exposed to space; it’s coated in a thin layer of amorphous silicon dioxide glass. Why wouldn’t you just expose the gold itself to the depths of space? Because it’s so soft and malleable, it’s highly susceptible to damage from even a mild or tiny impact. Whereas the beryllium is largely unaffected by micrometeoroid impacts, a thin gold coating would be, and would therefore be unable to maintain the smoothness necessary for the telescope’s operation without an additional layer of protection.
That’s where the final “coating atop the coating” comes in: of amorphous silicon dioxide glass. Although we typically associate mirrors with being made out of glass with some sort of coating on it, the function of the glass is very simple in this case: to be transparent to the light and to protect the gold. So yes, it’s gold coated, but then the gold itself needs to be protected with its own coating as well.
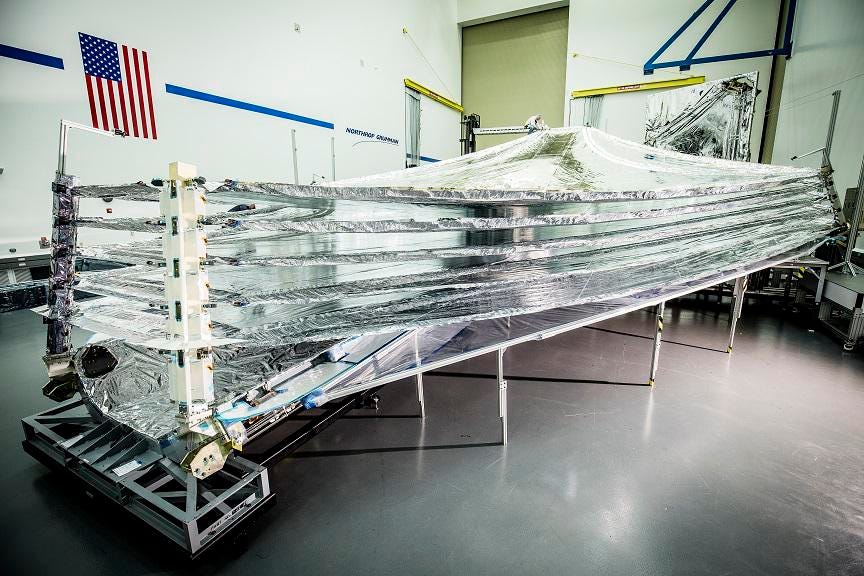
6.) The “telescope side” of James Webb will passively cool itself down to no higher than ~50 K: cool enough to make nitrogen liquify. The whole reason James Webb needs to be placed so far away from Earth, at the L2 Lagrange point instead of in low-Earth orbit like Hubble, is because it’s going to be passively cooled as never before. An enormous five-layered sunshield has been specially created for James Webb, reflecting as much of the sunlight away as possible and shielding the layer beneath it. If it were in low-Earth orbit, the infrared heat emitted by the Earth would prevent it from reaching the necessary low temperatures.
The diamond-shaped sunshield itself is enormous: 21.2 meters (69.5 feet) in the long dimension and 14.2 meters (46.5 feet) in the short dimension. Each layer has a “hot side” that faces the Sun and a “cold side” that faces the telescope. The outermost layer will, on its hot side, reach a temperature of 383 K, or 231 °F. By the time you get to the innermost layer, the hot side is only 221 K, or -80 °F, but the cold side is all the way down to 36 K, or -394 °F. So long as the telescope remains below ~50 K, it will be capable of operating as designed.
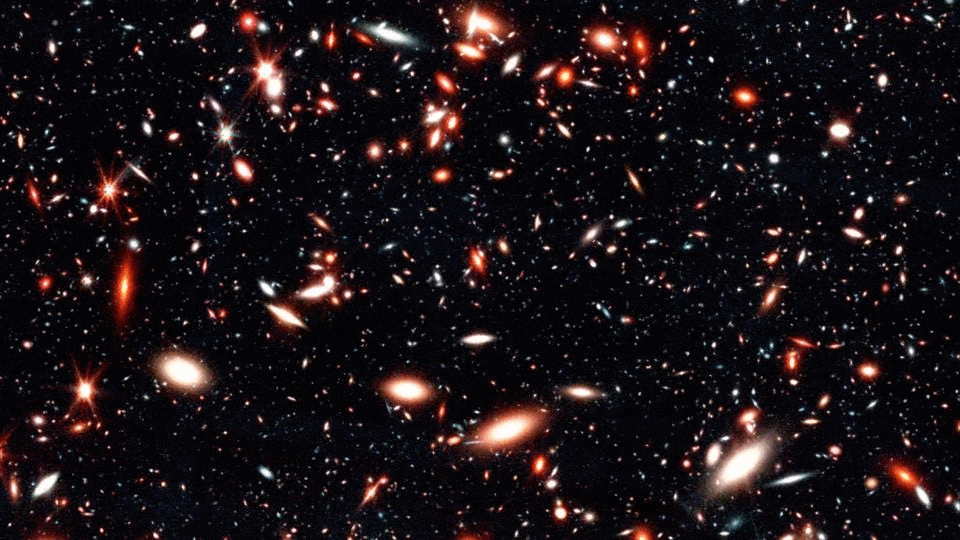
7.) With active, cryogenic cooling, Webb will get all the way down to ~7 K. The low temperatures reached by passive cooling, in the 36-to-50 K range, are completely sufficient for the operation of all of Webb’s near infrared instruments. This includes three of its four major science instruments: NIRCam (the near infrared camera), NIRSpec (the near infrared spectrograph), and the FGS/NIRISS (fine-guidance sensor/near infrared imager and slitless spectrograph). They’re all designed for operation at 39 K: well within the range of passive cooling.
But the fourth instrument, MIRI (the mid infrared imager), needs to be cooled even farther than passive cooling can get you, and that’s where the cryocooler comes in. Helium only becomes liquid at about 4 K, and so by attaching a liquid helium refrigerator to the MIRI instrument, Webb scientists can cool it down to the needed operating temperature: ~7 K. The longer the wavelength of light that you want to probe, the cooler you need to get your instruments, which is the primary reason for most of the design decisions that went into the James Webb Space Telescope.
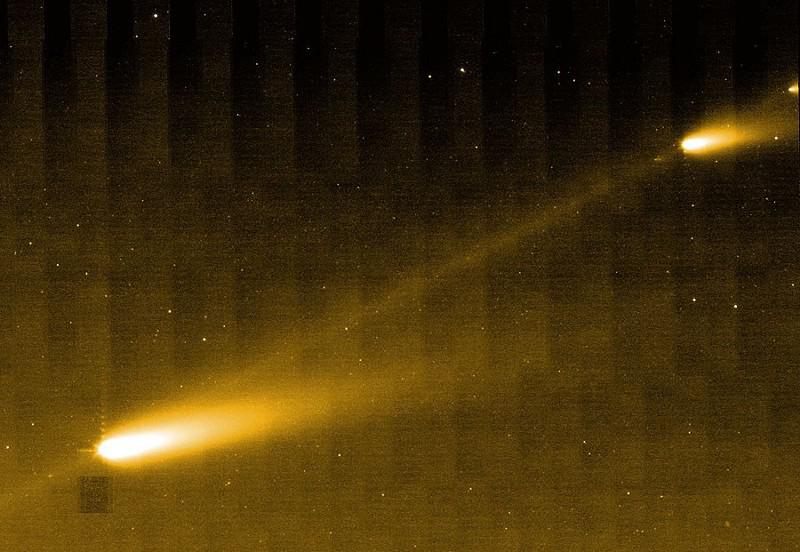
8.) Unlike NASA’s Spitzer, which transitioned to a “warm” mission when it ran out of coolant, James Webb should maintain its cold temperatures for its entire lifespan. The liquid helium that keeps James Webb actively cooled, in principle, should never run out; it’s a closed system. However, as anyone who’s ever worked in experimental physics can attest, leaks inevitably happen, no matter how well you safeguard against them. Designed for a 5.5-year mission, at minimum, with the possibility of a decade or longer under the most optimistic circumstances, Webb shouldn’t run out of its cryogenic coolant if it lives up to its design specifications.
However, there’s always the possibility that something will go wrong, and we won’t be able to actively cool the mid infrared imager sufficiently or for the entire mission, and that will eat into Webb’s sensitivities at progressively longer and longer wavelengths. (The same caveat applies to the near infrared instruments in the event of sunshield damage or inefficiencies.) The warmer the James Webb Space Telescope gets, the narrower its wavelength range it can probe will become.
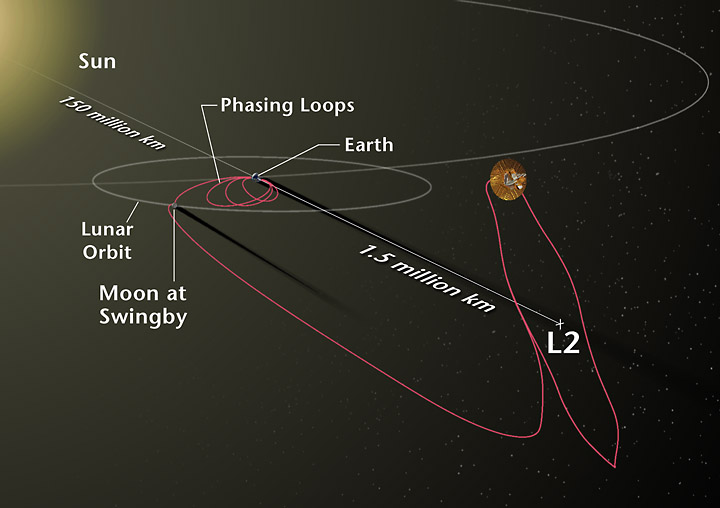
9.) When it runs out of fuel, its fate will be to permanently reside in a “graveyard orbit” around the Sun. Hubble, with an assist from four servicing missions, is still functioning more than three full decades after its launch. Webb, however, needs to use its fuel whenever it wants to do anything involving motion. That includes:
- to perform a burn to correct its course towards its destination at L2
- to perform orbital corrections to keep it in its orbit at L2
- to orient itself so that it points at its desired target
Fuel comes in a finite supply, and how much we have left for science operations depends entirely on the degree to which the launch puts Webb on its ideal trajectory toward its ultimate destination.
When it’s out of fuel, science operations end. However, we can’t just leave it out there to drift wherever it may go, as it would potentially endanger future missions destined for L2. Instead, just as we did for prior spacecraft sent to L2, like NASA’s WMAP satellite, we’ll send it to a graveyard orbit, where it will orbit the Sun for as long as there’s a Sun to orbit.
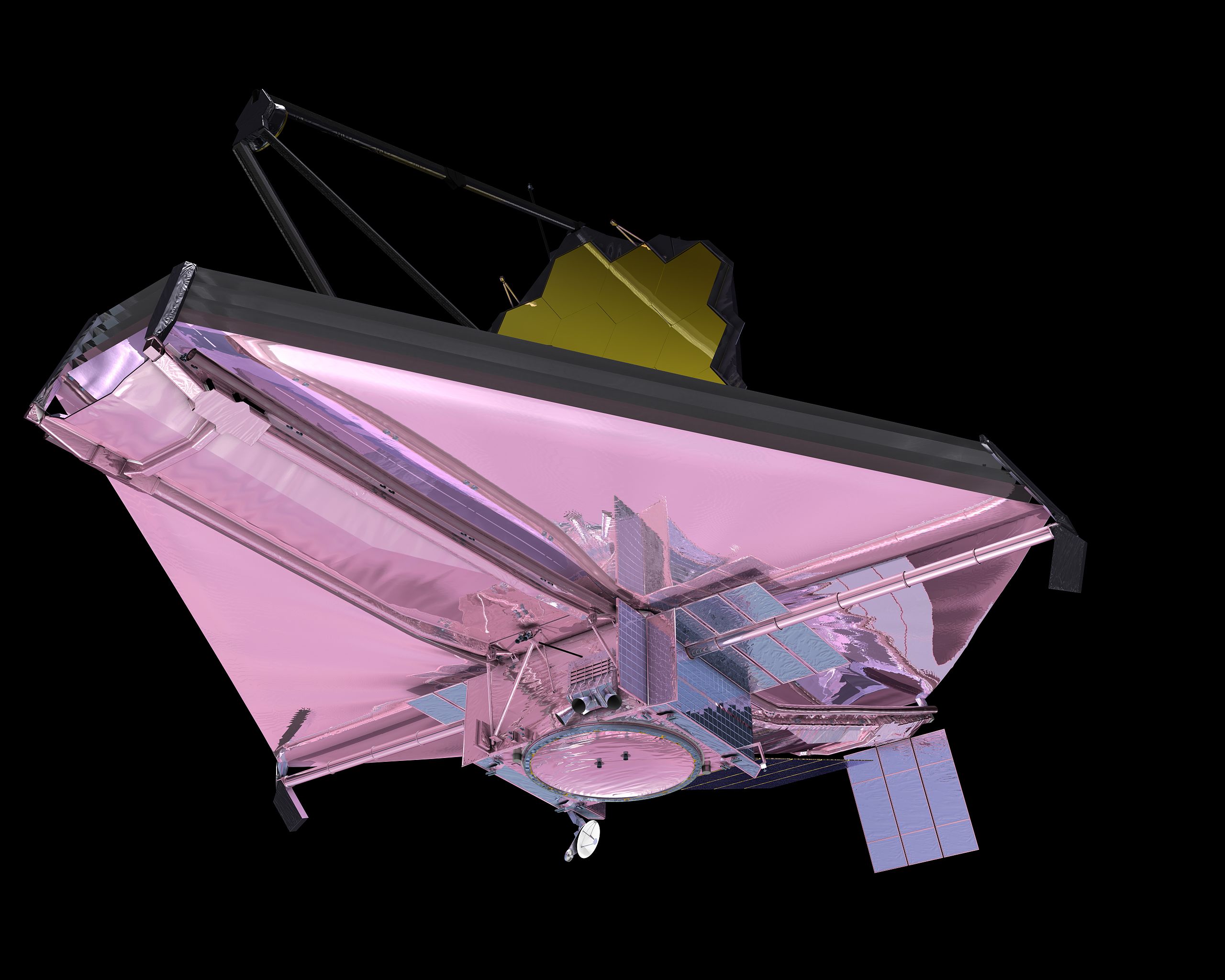
10.) Although it wasn’t designed to be serviced and upgraded, it could potentially be robotically refueled to extend its life. It seems a pity that Webb’s lifetime, after all of this effort, will be so finite. Sure, 5-to-10 years is enough time to learn a tremendous amount about the Universe, meeting a large number of ambitious science goals and opening ourselves up to possibility of serendipitous discoveries that we have perhaps not even yet imagined. But after all that we’ve been through with development and delays, it seems insufficient that James Webb will have a lifetime that’s cumulatively shorter than the full extent of its time here on Earth.
But there’s hope.
There’s a refueling port that, if we develop the right uncrewed technology, we could access. If we can get to L2, dock with James Webb, access the refueling port, and refuel it then the mission’s lifetime could be extended by a decade or more with each refuel. There have been rumors that the German Aerospace Center, DLR, could potentially perform exactly this type of operation before Webb reaches the end of its life, presumably in the early 2030s. If Webb works exactly as designed and is, as expected, fuel-limited, it might be the ultimate exercise in wasteful foolishness not to pursue that option.