Here’s how NASA’s James Webb Space Telescope will unveil the unknown Universe

- Despite all we’ve learned about the Universe, including what it looks like and what exists in it, there remain many cosmic unknowns.
- How do supermassive black holes form and grow early on? What were the very first stars like? What’s in the atmospheres of “super-Earth” planets?
- We don’t yet know the answers. But if James Webb succeeds as an observatory, it should teach us the answers to all of these questions, plus more.
Our modern perspective on the Universe is, simultaneously, both a triumph and a tragedy. The triumph is how, from our location around a random star inside a typical galaxy in a vast Universe, we’ve been able to learn so much about the cosmos we inhabit. We’ve discovered the laws that govern the Universe as well as the fundamental particles that make up reality. We’ve developed a cosmological model that can explain how the Universe came to be the way it is, with observations that take us from the present day back to the far reaches of the Universe: over 13 billion years ago and more than 30 billion light-years away in space. After countless generations of wondering, we finally know what the Universe looks like.
But there is tragedy in this story as well: all that remains unknown about the cosmos. We know that the normal matter we see under our currently known laws of physics are insufficient to explain the Universe on small and large scales; both dark matter and dark energy, at minimum, are required. We have an unresolved controversy over how fast the Universe is expanding. We’ve never seen the very first stars or galaxies. We’ve never measured the atmospheric contents of an Earth-sized exoplanet. We don’t know how supermassive black holes first formed. And the list goes on and on.
And yet, NASA’s newest flagship observatory, the James Webb Space Telescope, is poised to begin science operations in just a few months. Here’s what we all can’t wait to learn.

The very first stars. In the earliest moments of the hot Big Bang, the Universe formed individual protons and neutrons, and then those protons and neutrons fused together in the first few minutes to make the first heavier elements in the Universe. We believe we know, from a variety of lines of reasoning, what the ratios of those elements were before the Universe formed even a single star. By mass, the Universe was composed of:
- 75% hydrogen
- 25% helium-4
- ~0.01% helium-3
- ~0.01% deuterium (hydrogen-2)
- ~0.0000001% lithium-7
There seemed to be pretty much nothing else around. Of course, by the time we see stars of any variety, we already see that they possess some amount of oxygen and carbon: heavy elements, by astronomer’s standards. This indicates that the earliest stars we’ve seen were already preceded by an earlier, “first” generation of stars.
We’ve never seen an example of pristine stars before, and James Webb will be our best opportunity to do so. Its infrared eyes can peer back farther than any observatory, including Hubble, and should break the cosmic record for the earliest, most pristine stars ever seen. We have theories that they should be very massive and short lived. James Webb is expected to give us our first opportunity to spot and study them.

The formation of the first black holes. At the limits of today’s observations, we’ve spotted black holes that are as massive as about ~1 billion solar masses a whopping 13.2 billion years ago: when the Universe was merely ~5% of its present age. How did those early black holes get so massive so fast? It’s not impossible, but it certainly is a challenge for our current theories to explain what we see. We would need, for example, a “seed” black hole of about 10,000 solar masses to form just ~100 million years after the Big Bang, and it would then need to grow at the maximum rate that’s physically allowed for the entire time just to get there.
Either these black holes started off bigger than our theories expect, or they formed earlier than we realize, or they grow faster than we think they can. But that’s where James Webb should shed a remarkable amount of light on these dark objects. Because they accelerate the matter accreting onto them, supermassive black holes can often be seen in radio wavelengths, identifiable as quasars. With its infrared eyes, Webb will be able to pick out the host galaxies that house these quasars, allowing us to match them up at these great cosmic distances for the first time. If we want to understand how black holes grow in the young Universe, there’s no better tool than Webb for finding out.
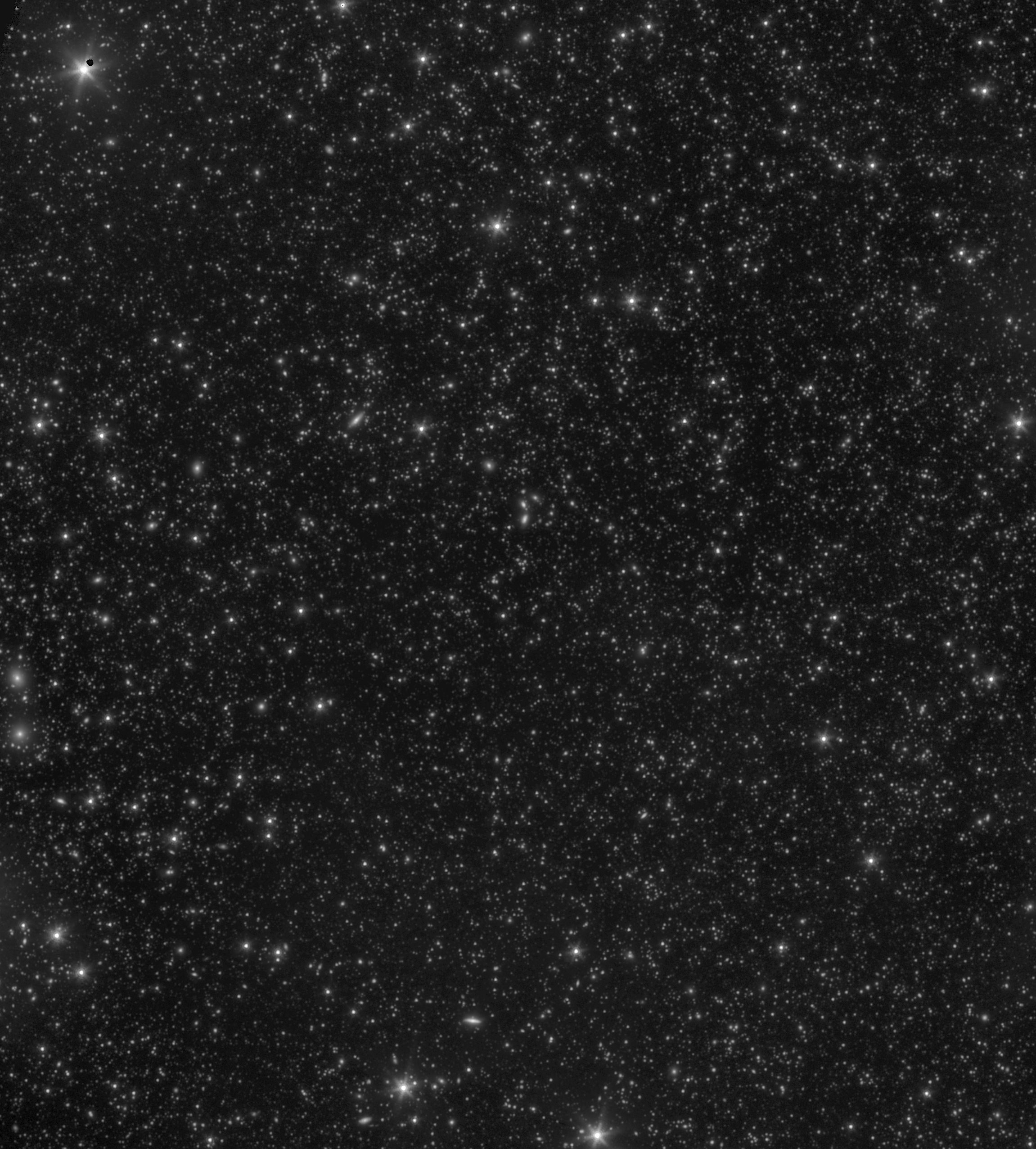
The clustering of galaxies across cosmic time. Do you see the image above? What looks like a bunch of stars silhouetted against the black backdrop of space isn’t stars at all; rather, each “dot” in this image is its own galaxy. NASA’s Spitzer, which was our flagship infrared observatory when it was launched in 2003, was able to see through the light-blocking dust that obscured many of these galaxies in optical wavelengths. Spitzer originally embarked on an observing program called SEDS: the Spitzer Extended Deep Survey, which grabbed a full square-degree of sky, and then the follow-up, S-CANDELS, went even deeper.
The results of that revealed the non-random clustering of galaxies, helping us to understand the gravitational history, growth, and evolution of our Universe, while also revealing another line of evidence for the necessity of dark matter. As part of its first year of science scheduled during its mission lifetime, the James Webb Space Telescope will map 0.6 square degrees of sky — about the area of three full Moons — with its infrared instruments, revealing galaxies that even Hubble could not see. If we want to see how galaxies grow and evolve across cosmic time, as well as how they cluster, to infer the dark matter web holding the cosmos together, Webb will give us an unprecedentedly valuable piece of data.
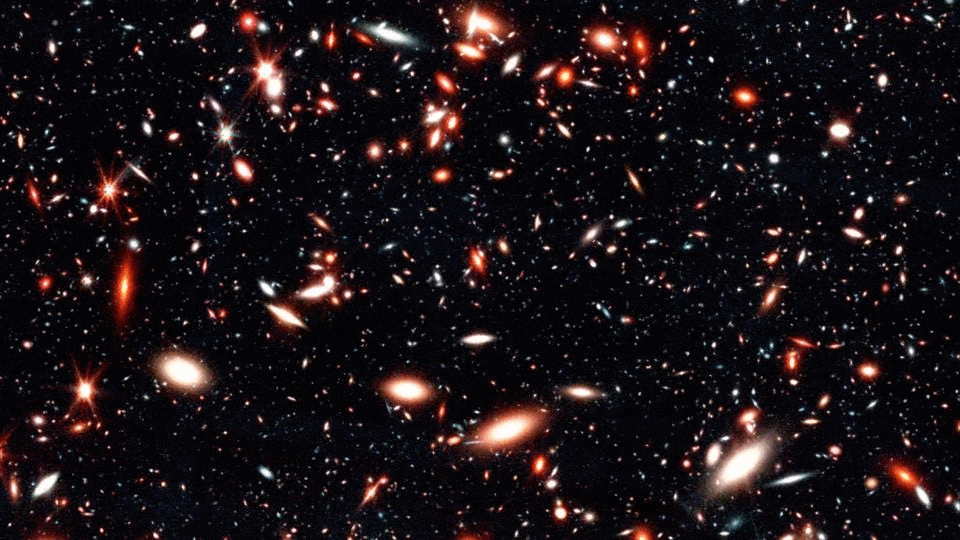
What’s out there in the deepest depths of space? If we look back across cosmic time with Hubble, we quickly run into two fundamental limitations. One comes from the expanding Universe itself, which stretches the wavelength of the light that gets emitted. While the hottest, youngest stars emit copious amounts of ultraviolet light, the expansion of the Universe shifts that light all the way out of the ultraviolet, through the optical and into the infrared by the time it arrives at our eyes. A “normal” telescope simply won’t see objects beyond a certain distance.
The second limitation is that there are neutral atoms in intergalactic space that absorb light, at least for the first ~550 million years or so of our cosmic history. Both of these factors limit what our current deepest telescopes, like Hubble, have been able to see.
But NASA’s James Webb Space Telescope will take us well beyond those current limitations, as its capabilities to go far into the infrared — to maximum wavelengths some 15 times longer than Hubble can probe — allowing us to both capture the shifted light and to see light that was initially infrared, which can evade the prevalent neutral atoms. As a result, we’ll find the most distant galaxies of all-time, learn how quickly and abundantly they formed stars, and be able to characterize them as never before, too.
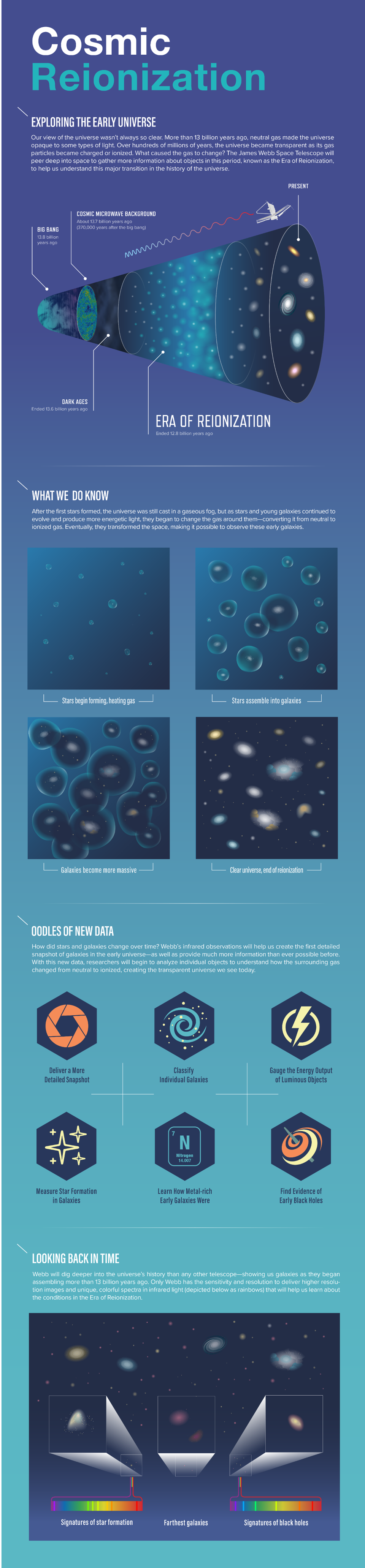
The physics of reionization. It took approximately 380,000 years for the Universe to expand and cool enough so that neutral atoms could stably form. But then it took another 550,000,000 years before those atoms became reionized, allowing visible light to travel freely through the Universe without getting absorbed. Hubble has only ever observed perhaps two or three galaxies beyond that limit, all along lines of sight where reionization occurred serendipitously earlier than average.
But that’s a clue! Reionization didn’t happen all at once, but was rather a gradual process that occurred in bursts. As stars form, they emit ultraviolet radiation, which ionizes the neutral atoms they encounter. Early on, those newly formed ions and electrons can still recombine, but later on, the Universe has expanded sufficiently that they no longer encounter one another frequently enough. We have simulations that tell us how we expect the process of reionization to play out, but only James Webb will be able to probe the galaxy-black hole connection, and collect the data to show us:
- how individual galaxies formed and evolved
- how much energy gets outputted by these luminous objects
- how rich in heavy elements these first galaxies were
- how rich in stars and what the current star-formation rates of these galaxies are
Right now, the pre-reionization epoch is known as “the cosmic dark ages.” But Webb, for the first time, will light it up for all to see.
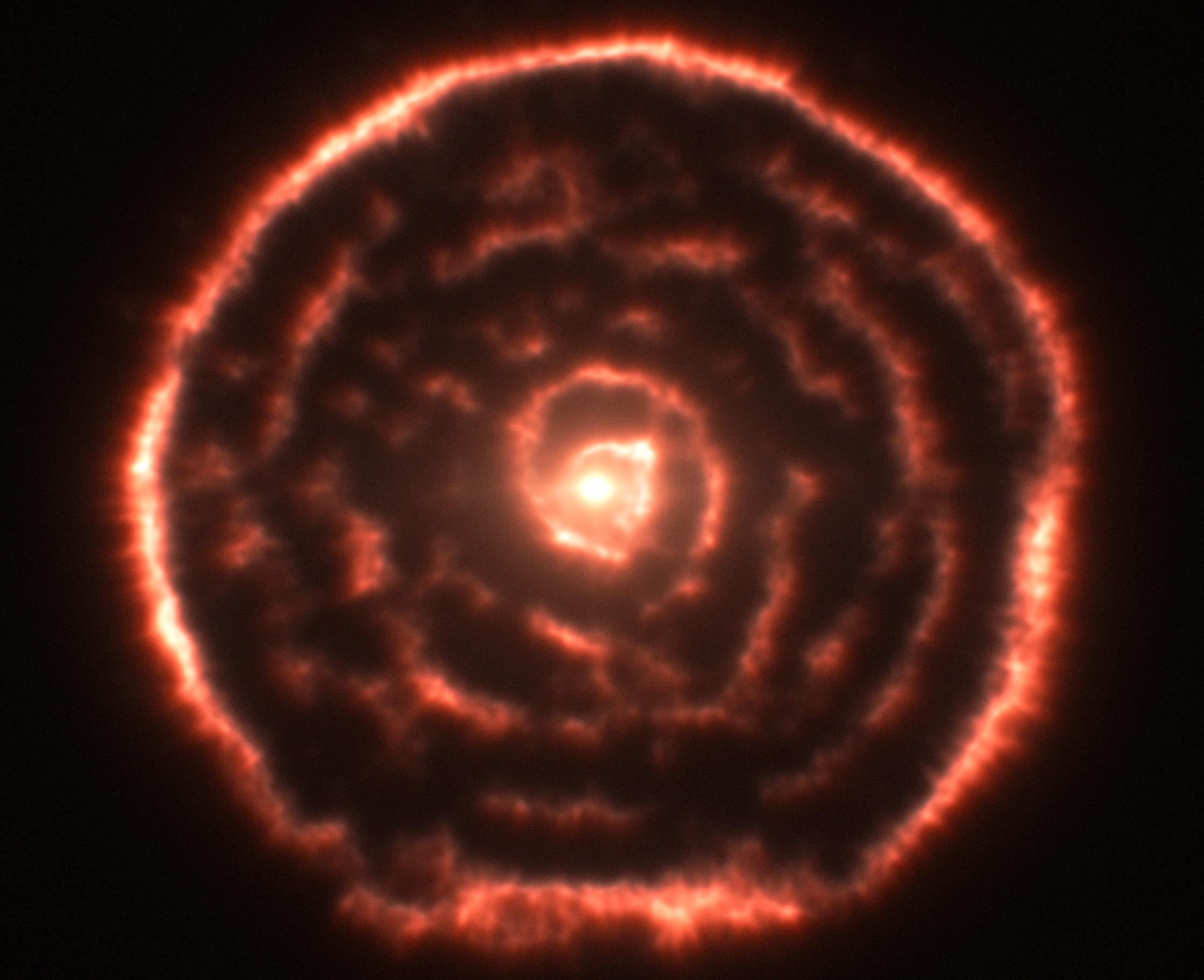
What enriches the Universe? The earliest stars we’ve seen are what we know of as metal-poor. Compared to our Sun, some of them contain only 1% of the total amount of heavy elements that we do, while others have as little as 0.01% or even less. The stars that formed the earliest and in the most pristine environments tend to be the closest to “metal-free” as we’ve ever come, but science isn’t just about finding the most extreme examples of what’s out there; it’s also about learning how the Universe came to be the way it is now.
That’s one of the greatly underappreciated places where Webb will truly shine: by studying interstellar dust. It’s actually the dust between the stars that will inform us about how two specific populations of stars — aging, massive stars and supernovae — enrich the Universe with heavy elements. It’s generally recognized that stars in their death throes are what create the heavy elements that populate the cosmos, but research is still being done into which elements are produced where, and in what proportion.
For example, stars on the asymptotic giant branch fuse carbon-13 with helium-4, producing neutrons, and the absorption of those neutrons builds up the elements in the periodic table. Stars going supernova also produce neutrons, and the absorption of those neutrons build up elements as well. But which elements come from which processes, and in which fractions? Webb will help answer the quantitative part of this question, whose answer has eluded us for so long.

How do planetary systems form? In recent years, a combination of two different types of ground-based observing have shown us the details in newly forming protoplanetary systems as never before. ALMA, the Atacama Large Millimeter/submillimeter Array, has shown us these protoplanetary disks in unprecedented detail, revealing a rich structure, including gaps that indicate where young planets have swept up the disk material, and even the formation of circumplanetary disks, in some cases. Meanwhile, infrared observatories have imaged extended, outer disks, revealing their structure as well.
Where James Webb will shine, however, is in those presently elusive innermost regions, as it will be our most powerful space-based, diffraction-limited telescope ever. Most of the work done so far can determine the structure of these disks out where the gas giants in our Solar System are and beyond; James Webb will be able to measure these disks in the region where our rocky, terrestrial, and innermost planets have formed, and it may even be able to find structures that are on scales as small as ~0.1 astronomical units, or a quarter of the distance from Mercury to the Sun.
Particularly around newly forming stars that are relatively close to us, the James Webb Space Telescope will reveal structures around new stars that we’ve only dreamed of uncovering. It’s one of the biggest revolutions in exoplanet sciences, but not the biggest, that Webb will bring.

Direct exoplanet imaging. When it comes to most of the planets that we’ve discovered, it might surprise you to learn that we’ve never actually “seen” them. We either measure the wobble of the parent star owing to the planet’s gravitational influence, revealing the planet’s mass and period, or we measure the periodic blocking of light that occurs when the planet in question transits in front of the stellar disk, revealing its radius and period. But the only planets we are currently capable of imaging are:
- well-separated from the parent star
- large enough to either reflect enough starlight or emit their own infrared light
- bright enough compared to the parent star to be seen in the parent star’s glare
As a result, most directly imaged planets are super versions of Jupiter: large, distant, and seen in relatively close systems where a coronagraph could be used to block the light from the parent star.
From its location in space, with its infrared eyes, and with its 6.5-meter-diameter primary mirror, James Webb will blow everything else away. We’re talking about the smallest, closest planets ever: down to about 1.5 times the size of Earth around Sun-like stars, and possibly down to Earth-sized worlds around red dwarfs. If we get very, very lucky, we might get our first signs of a world with varying clouds, seasons, and possibly even oceans and continents. Only with James Webb will these observations be possible.

Measuring the atmospheres of the smallest planets ever. But this, in my opinion, is the realm that offers the biggest possibility of a truly revolutionary breakthrough. When a planet passes in front of its parent star, what happens? Yes, the planet blocks a portion of the star’s light, causing the characteristic dimming — or flux dips — that we associate with a classical transit. But something else also occurs, if the planet has an atmosphere: A portion of the star’s light filters through the atmosphere, where atoms and complex molecules exist. The “filtered” portion of the star’s light will therefore get absorbed at particular wavelengths. If we can measure those wavelengths, we can infer what molecules exist in that planet’s atmosphere.
Could we find molecular oxygen, carbon dioxide, or perhaps complex biomolecules?
Yes to all of the above. If they’re present, and they absorb at wavelengths to which NASA’s James Webb Space Telescope is sensitive, we have a chance to reveal an inhabited planet for the very first time. We don’t know whether any of the planets that Webb will be capable of measuring the atmospheres of are actually inhabited or not. But this is the most exciting type of science: the kind where we’re looking as we never have before. If we detect a positive signal, it will change our view of the Universe forever. It’s hard to ask for more than that.

All of this, of course, leaves out the grandest possibility of all. We know where the frontiers of our knowledge are today; we can walk right up to them and peer over the ledge into the sea of vast cosmic unknowns. NASA’s James Webb Space Telescope will push those frontiers in a variety of ways, and we can predict what sort of incremental progress will be made, and what present unknowns will be revealed by gaining this information that eludes us at present. But what we can’t predict is what’s out there that we presently don’t have any clues about. We don’t know what sorts of remarkable discoveries we’ll be able to make simply because we’re looking at the Universe as we never have before.
That’s, arguably, the most important piece of doing science: the ability to open up what we call “discovery potential.” We know some of what’s out there, and that’s led us to some excellent expectations for what we anticipate that we’re going to find. But what about the things that are out there that we presently have no hints of? Until we look, we don’t know. Perhaps the search was best summed up by Edwin Hubble, but his sentiments apply precisely to the Webb Telescope as well.
“With increasing distance, our knowledge fades, and fades rapidly. Eventually, we reach the dim boundary—the utmost limits of our telescopes,” Hubble said. “There, we measure shadows, and we search among ghostly errors of measurement for landmarks that are scarcely more substantial. The search will continue. Not until the empirical resources are exhausted, need we pass on to the dreamy realms of speculation.”