Why gravitational waves are the future of astronomy
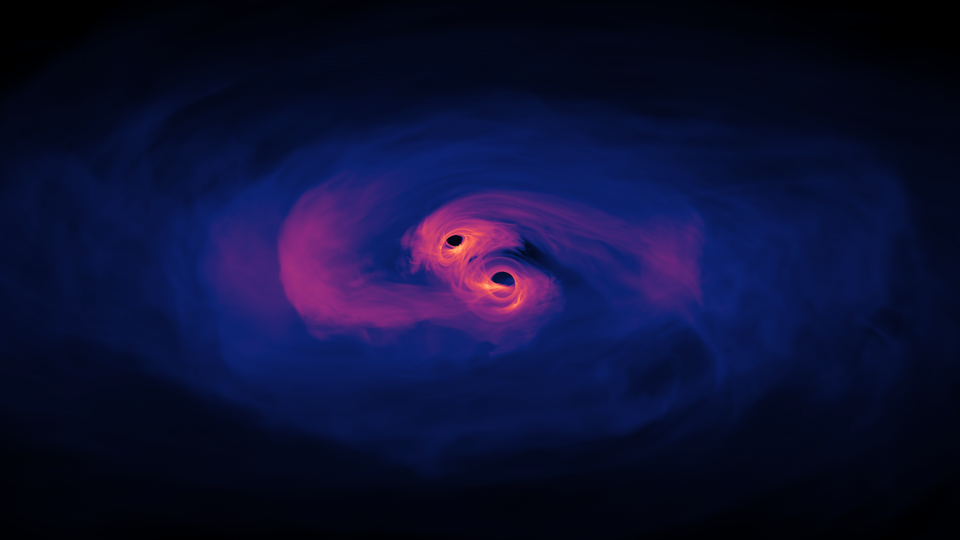
- Although gravitational waves were an extractable prediction from Einstein’s General Relativity all the way back in 1915, it took 100 years for humanity to successfully detect them.
- Today, we’ve detected merging black holes, merging neutron stars, and neutron stars merging with black holes via gravitational waves, but so much more is still to come.
- A whole series of new detections will be enabled with upcoming technology, ushering in a new era of astronomy for us all, and expanding the definition of what “astronomy” actually entails.
It was over 100 years ago that Einstein put forth, in its final form, the General theory of Relativity. The old Newtonian conception of gravitation — where two massive objects attracted one another, instantaneously, with a force proportional to their masses and inversely proportional to the square of the distance between them — disagreed with both the observations of Mercury’s orbit and the theoretical requirements of special relativity: where nothing could travel faster than light, not even the force of gravity itself.
General Relativity replaced Newtonian gravity by instead treating spacetime as a four-dimensional fabric, where all the matter and energy traveled through that fabric: limited by the speed of light. That fabric wasn’t simply flat, like a Cartesian grid, but rather had its curvature determined by the presence and motion of matter and energy: matter and energy tells spacetime how to curve, and that curved spacetime tells matter and energy how to move. And whenever an energy-containing object moved through curved space, one inevitable consequence is that it would emit energy in the form of gravitational radiation, i.e., gravitational waves. They’re everywhere in the Universe, and now that we’ve begun to detect them, they’re about to open up the future of astronomy. Here’s how.

The first two things you need to know, in order to understand gravitational wave astronomy, is how gravitational waves are generated and how they affect quantities that we can observe in the Universe. Gravitational waves are created whenever an energy-containing object passes through a region where the spacetime curvature changes. This applies to:
- masses orbiting other masses,
- rapid changes in a spinning or collapsing object,
- the merger of two massive objects,
- and even a set of quantum fluctuations that were created during the inflationary epoch that preceded and set up the hot Big Bang.
In all of these cases, the energy distribution within a particular region of space changes rapidly, and that results in the production of a form radiation inherent to space itself: gravitational waves.
These ripples in the fabric of spacetime travel at precisely the speed of light in a vacuum, and they cause space to alternately compress-and-rarify, in mutually perpendicular directions, as the peaks and troughs of the gravitational waves pass over them. This inherently quadrupolar radiation affects the properties of the space that they pass through, as well as all objects and entities within that space.
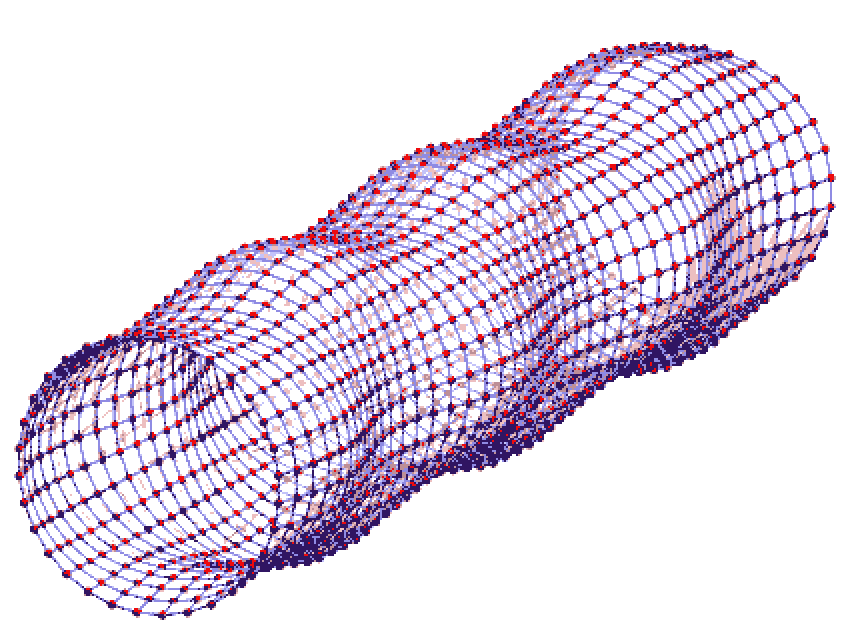
If you want to detect a gravitational wave, you need some way to be sensitive to both the amplitude and frequency of the wave you’re searching for, and you also need to have some way to detect that it’s affecting the region of space you’re measuring. When gravitational waves pass through a region of space:
- they come in with a specific direction, where space “compresses” and “rarifies” in the two mutually perpendicular directions to its propagation,
- they compress-and-rarify with a particular amplitude, which tells you how sensitive you need to be to changes in things like “distance” or “light-travel-time” in order to see them,
- and they oscillate at a particular frequency, where that frequency is determined only by the source that generated the gravitational waves of interest and the amount that the expansion of the Universe has stretched the gravitational waves as they’ve propagated through the Universe.
Numerous detection schemes have been proposed, including vibrating bars that would be sensitive to the oscillatory motion of a passing gravitational wave, pulsar timing that would be sensitive to oscillatory changes of gravitational waves that passed through the pulse’s line-of-sight with respect to us, and reflected laser arms that span different directions, where the relative changes between the multiple path-lengths would reveal the evidence of a gravitational wave as it passed through.

The last of these is precisely the first — and thus far, the only — method by which we’ve ever successfully detected gravitational waves. Our first such detection came on September 14, 2015 and represented the inspiral and merger of two black holes of 36 and 29 solar masses, respectively. As they merged together, they formed a final black hole of only 62 solar masses, with the “missing” three solar masses getting converted into pure energy, via E = mc², in the form of gravitational waves.
As those waves passed through planet Earth, they alternately compressed-and-rarified our planet by less than the width of a blade of grass: a minuscule amount. However, we had two gravitational wave detectors — the LIGO Hanford and LIGO Livingston detectors — that each consisted of two perpendicular laser arms, 4 km long, that reflected lasers back-and-forth over a thousand times before the beams were brought back together and recombined.
By observing the periodic shifts in the interference patterns created by the combined lasers, which themselves were caused by the passing gravitational waves through the space that the laser light was traveling through, scientists were able to reconstruct the amplitude and frequency of the gravitational wave that passed through. For the first time, we’d captured these now-infamous ripples in spacetime.
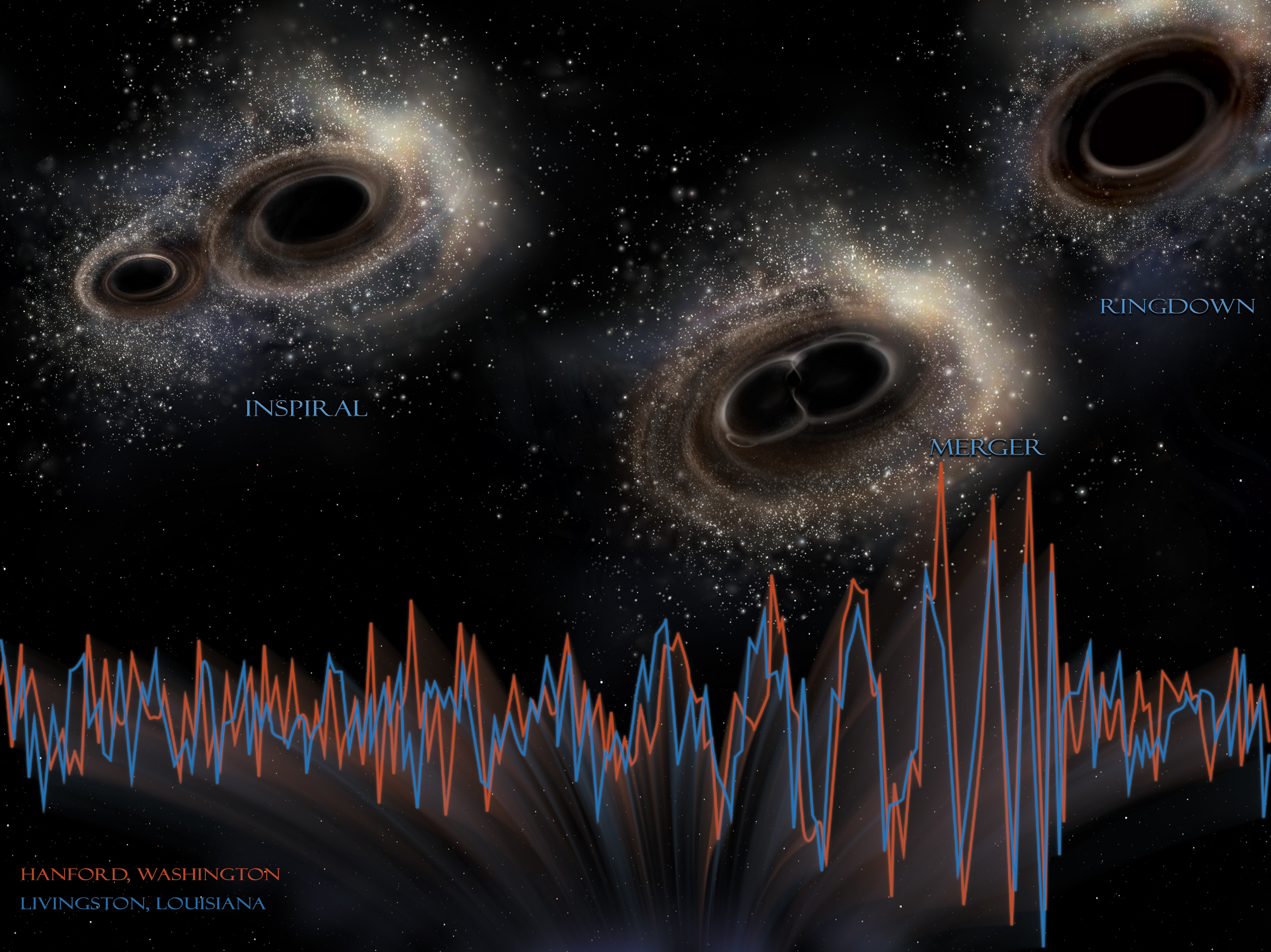
Since that time, the twin LIGO detectors have been joined by two other ground-based laser interferometer gravitational wave detectors: the Virgo detector in Europe, and the KAGRA detector in Japan. By the end of 2022, all four detectors will combine to produce an unprecedented gravitational wave detector array, allowing them to be sensitive to lower-amplitude gravitational waves originating from across more locations on the sky than ever before. Later this decade, they’ll be joined by a fifth detector, LIGO India, which will increase their sensitivity even further.
You have to realize that every gravitational wave that passes through Earth comes in with a specific orientation, and only the orientations that cause substantial shifts in both perpendicular laser-arms of an individual detector can lead to a detection. The twin LIGO Hanford and LIGO Livingston detectors are specifically oriented for redundancy: where the angles the detectors are at, relative to one another, is precisely compensated for by the curvature of the Earth. This choice ensures that a gravitational wave that appears in one detector will also appear in the other, but the cost is that a gravitational wave that’s insensitive to one detector will also be insensitive to the other. In order to get better coverage, more detectors with a diversity of orientations — including detectors sensitive to orientations that LIGO Hanford and LIGO Livingston will miss — are necessary to win the Pokémon-esque game of “catching them all.”

But even with up to five detectors, with four independent orientations between them, our gravitational wave capabilities will still be limited in two important ways: in terms of amplitude and frequency. Right now, we have somewhere in the ballpark of ~100 gravitational wave events, total, but all of them are from relatively low-mass, compact objects (black holes and neutron stars) that have been caught in the final stages of inspiraling and merging together. In addition, they’re all relatively nearby, with black hole mergers extended out a few billion light-years and neutron star mergers reaching perhaps a couple of million light-years. So far, we’re only sensitive to the black holes that are around 100 solar masses or under.
Again, the reason is simple: gravitational field strengths increase the closer you get to a massive object, but the closest you can get to a black hole is determined by the size of its event horizon, which is primarily determined by a black hole’s mass. The more massive the black hole, the larger its event horizon, and that means the greater the amount of time it takes for any object to complete an orbit while still remaining outside the event horizon. The lowest-mass black holes (and all of the neutron stars) allow for the shortest orbital periods around them, and even with thousands of reflections, a laser arm that’s only 3-4 km long isn’t sensitive to longer time periods.
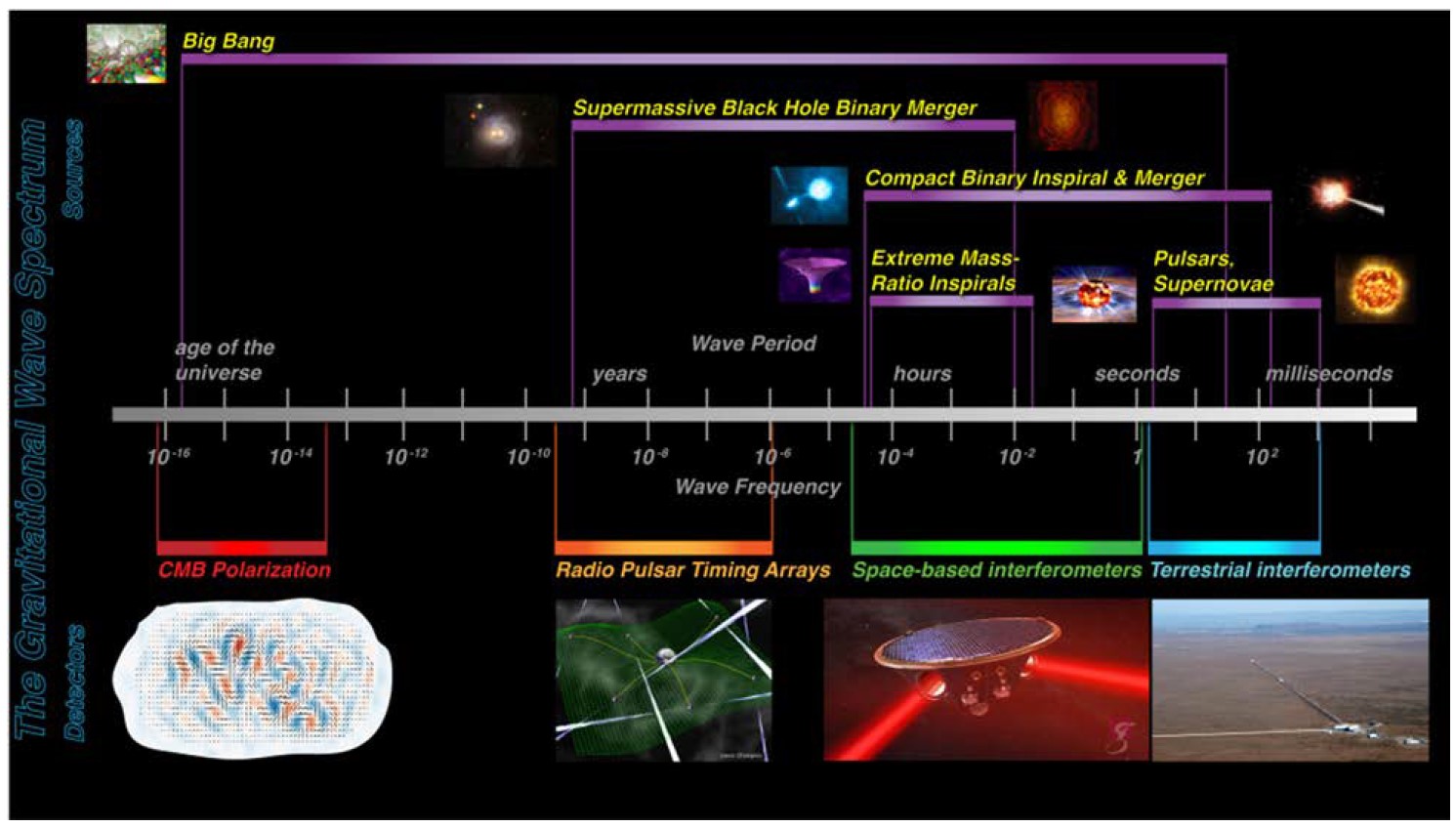
That’s why, if we want to detect the gravitational waves emitted by any other sources, including:
- more massive black holes, like the supermassive ones found at the centers of galaxies,
- less compact objects, like orbiting white dwarfs,
- a stochastic background of gravitational waves, caused by the cumulative sum of all the ripples generated by all of the supermassive black hole binaries whose waves constantly pass us by,
- or the “other” background of gravitational waves: the ones left over from cosmic inflation that still persist throughout the cosmic today, 13.8 billion years after the Big Bang,
we need a new, fundamentally different set of gravitational wave detectors. The ground-based detectors we have today, despite how fabulous they truly are in their realm of applicability, are limited in amplitude and frequency by two factors that cannot be easily improved. The first is the size of the laser arm: if we want to improve our sensitivity or the frequency range that we can cover, we need longer laser arms. With ~4 km arms, we’re already seeing just about the highest-mass black holes we can; if we want to probe either higher masses or the same masses at greater distances, we’d need a new detector with longer laser arms. We might be able to build laser arms perhaps ~10 times as long as the current limits, but that’s the best we’ll ever be able to do, because the second limit is set by planet Earth itself: the fact that it’s curved along with the fact that tectonic plates exist. Inherently, we can’t build laser arms beyond a certain length or a certain sensitivity here on Earth.

But that’s okay, because there’s another approach that we should begin taking in the 2030s: creating a laser-based interferometer in space. Instead of being limited by either the fundamental seismic noise that cannot be avoided as the Earth’s crust moves atop the mantle, or by our ability to construct a perfectly straight tube given the curvature of the Earth, we can create laser arms with baselines hundreds of thousands or even millions of kilometers long. This is the idea behind LISA: the Laser Interferometer Space Antenna, scheduled to be launched in the 2030s.
With LISA, we should be able to achieve pristine sensitivities at lower frequencies (i.e., for longer gravitational wave wavelengths) than ever before. We should be able to detected black holes in the thousands-to-millions of solar mass range, as well as highly mismatched black hole mass mergers. Additionally, we should be able to see sources that LIGO-like detectors will be sensitive to, except in much earlier stages, giving us months or even years of notice to prepare for a merger event. With enough such detectors, we should be able to pinpoint precisely where these merger events are going to occur, enabling us to point our other equipment — particle detectors and electromagnetically-sensitive telescopes — to the right location right at the critical moment. LISA, in many ways, will be the ultimate triumph for what we currently call multi-messenger astronomy: where we can observe light, gravitational waves, and/or particles originating from the same astrophysical event.
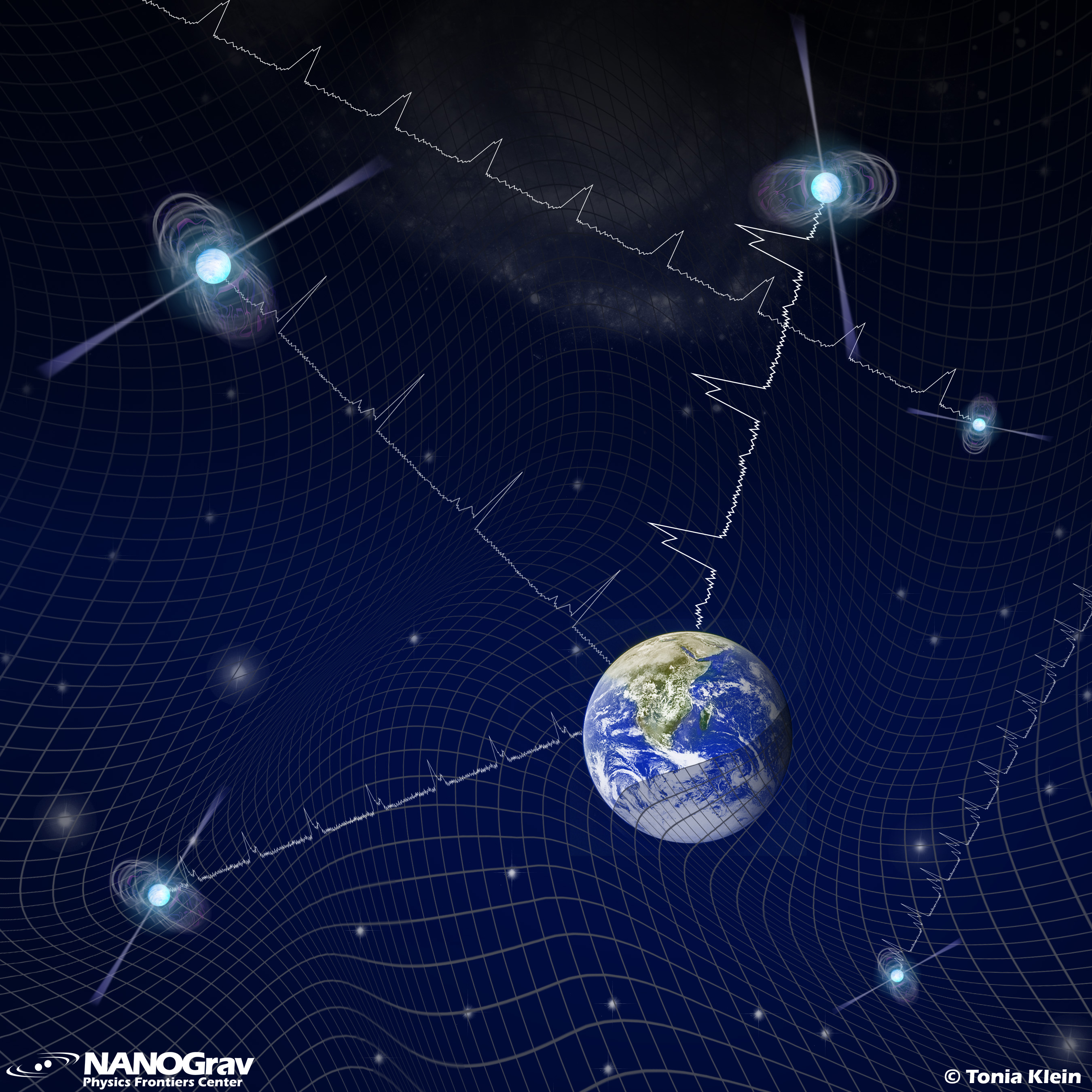
But for even longer-wavelength events, generated by:
- billion-solar-mass black holes orbiting one another,
- the sum of all supermassive black hole binaries in the Universe,
- and/or the gravitational wave background imprinted by cosmic inflation,
we need even longer baselines to probe. Fortunately, the Universe delivers us exactly such a way to do it, naturally, simply by observing what’s out there: precise, accurate, natural clocks, in the form of millisecond pulsars. Found all throughout our galaxy, including thousands and tens-of-thousands of light-years away, these natural clocks emit precisely-timed pulses, hundreds of times per second, and are stable on the timescales of years or even decades.
By measuring the pulse periods of these pulsars precisely, and by stitching them together into a continuously-monitored network, the combined timing variations seen across pulsars can reveal these signals that no currently proposed human-created detector could uncover. We know there ought to be many supermassive black hole binaries out there, and the most massive such pairs could even be detected and pinpointed individually. We have lots of circumstantial evidence that an inflationary gravitational wave background should exist, and we can even predict what its gravitational wave spectrum should look like, but we do not know its amplitude. If we’re lucky in our Universe, in the sense that the amplitude of such a background is above the potentially detectable threshold, pulsar timing could be the Rosetta Stone that unlocks this cosmic code.
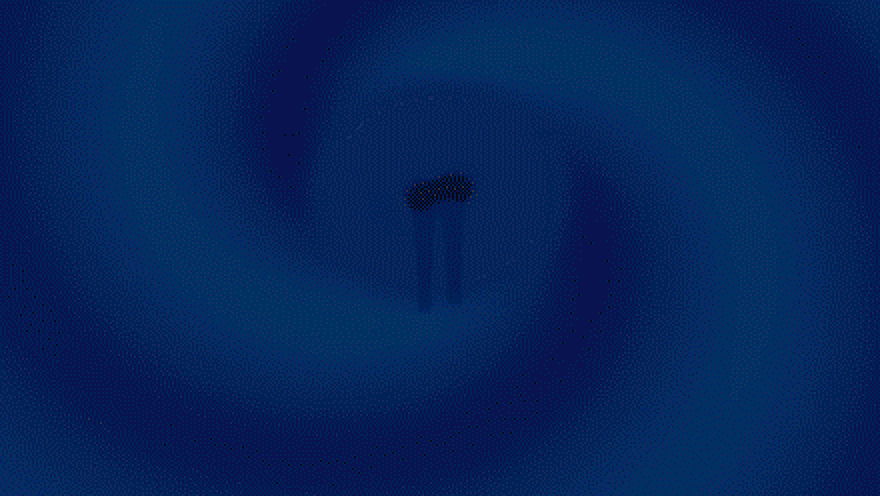
Although we firmly entered the era of gravitational wave astronomy back in 2015, this is a science that’s still in its infancy: much like optical astronomy was back in the post-Galileo decades of the 1600s. We only have one type of tool for successfully detecting gravitational waves right now, can only detect them in a very narrow frequency range, and can only detect the closest ones that produce the largest-magnitude signals. As the science and technology underlying gravitational wave astronomy continues to progress, however, to:
- longer-baseline terrestrial detectors,
- space-based interferometers,
- and increasingly sensitive pulsar timing arrays,
we’re going to reveal more and more of the Universe as we’ve never seen it before. In combination with cosmic ray and neutrino detectors, and being joined by traditional astronomy from across the electromagnetic spectrum, it’s only a matter of time before we achieve our first trifecta: an astrophysical event where we observe light, gravitational waves, and particles all from the same event. It might be something unexpected, like a nearby supernova, that delivers it, but it might also come from a supermassive black hole merger from billions of light-years away. One thing that’s certain, however, is that whatever the future of astronomy looks like, it’s definitely going to need to include a healthy and robust investment in the new, fertile field of gravitational wave astronomy!