We understand planets better than ever, and that’s why Pluto still isn’t one

- Discovered in 1929, Pluto was known as our solar system’s 9th planet for nearly 80 years.
- In 2006, the International Astronomical Union controversially defined the word planet, excluding Pluto forever.
- Today, we know so much more about worlds near and far, and Pluto just doesn’t measure up in all ways except one.
From 1929 until 2006, Pluto lived in the imagination of children and adults alike as the ninth and outermost planet in our solar system. Until 1978, with the discovery of its giant moon, Charon, it was the only known large object in our solar system that orbited beyond the reach of Neptune. And yet, throughout the 1990s and 2000s, a tremendous number of objects were discovered — including planets orbiting stars other than our Sun and a wide variety of Kuiper belt objects both large and small — that compelled us to rethink just what it meant for an object to be considered a planet.
In 2006, with only a small fraction of the general assembly in attendance, the International Astronomical Union put forth three criteria that an object needed to meet in order to be considered a planet:
- It must be massive enough to pull itself into hydrostatic equilibrium, where gravitation and rotation determine its overall shape.
- It must orbit the Sun and the Sun alone, eliminating any satellite worlds such as moons.
- It must “clear its orbit,” meaning that, over solar system-like timescales, there are no other comparably-massed objects that share its orbit.
Rather than adding in additional planets such as Ceres and Eris, this move instead demoted Pluto, stripping it of its planetary status. This definition remains controversial even today, but alternatives that draw a dividing line with Pluto on the other side are all scientifically indefensible. Here’s why.
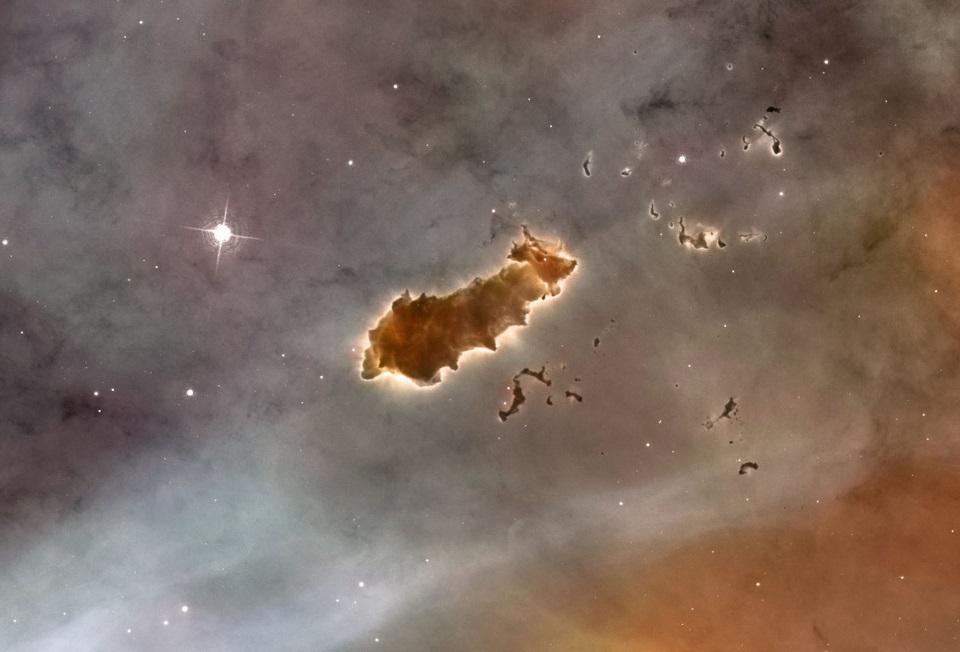
Credit: NASA, ESA, N. Smith, UC Berkeley, and the Hubble Heritage Team (STScI/AURA)
Normally, discussions about what is or isn’t a planet start from entirely the wrong place: an arbitrary definition that’s based on some idea of what a defining “planetary” characteristic is. Instead of thinking we know something about planets from the start — an “I know it when I see it” type of definition — we should be starting with what physically occurs when stars, planets, and all other sorts of objects form. To uncover that, we have to look inside the regions where this type of formation actually occurs: into the nebulae where active, new stars are actively forming.
Inside these massive, dusty, and gas-rich regions, the same series of events always occurs. First, a massive cloud of matter begins to collapse under the weight of its own gravitation. As gravitational collapse occurs, the regions that attract the most matter into them the fastest begin growing ever more rapidly. Since gravitation is a runaway process, it’s the locations of greatest density that collect the most matter and grow the fastest, and hence, will be the first locations to trigger the formation of new stars. Because of how large these regions are and how much angular momentum is contained inside of them, we don’t simply form one ultra-massive star, but rather hundreds, thousands, or even greater numbers of stars all at once.
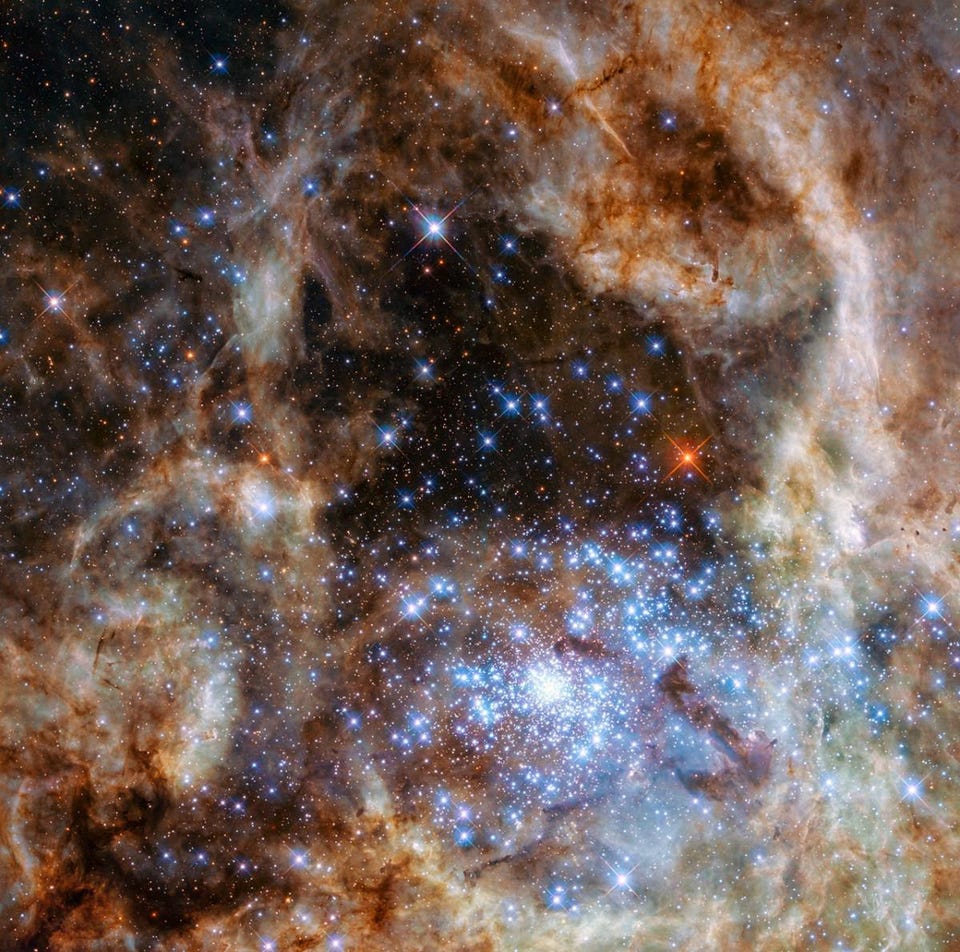
Credit: NASA, ESA, and P. Crowther (University of Sheffield)
For a long time, we only knew parts of this story. We could see the dark nebulae where this neutral matter was located, and where stars will form in the relatively near cosmic future. We could see, during the active stages of star formation, the surrounding ionized (mostly hydrogen) gas that emits light once there’s a sufficient amount of ultraviolet radiation inside from new, young stars. And finally, when sufficient amounts of that material evaporates away, we can see the exposed new stars from inside: these open star clusters filled with hundreds, thousands, or even greater numbers of new stars.
With the advent of high-resolution, multi-wavelength astronomy, however, we’ve been able to peer inside these once obscure regions to shed light on what’s occurring in these environments. Today, a rich story has been revealed. Every star-forming region not only has massive, growing clumps that will become stars with their own solar systems, but also a tremendous number of failed stars and solar systems: regions where the most massive object never becomes heavy enough to ignite nuclear fusion in its own core. Amid all the new stars lie even greater numbers of brown dwarfs and also less massive objects, around the physical size of Jupiter (and smaller), that simply didn’t grow big enough quickly enough to become stars on their own.
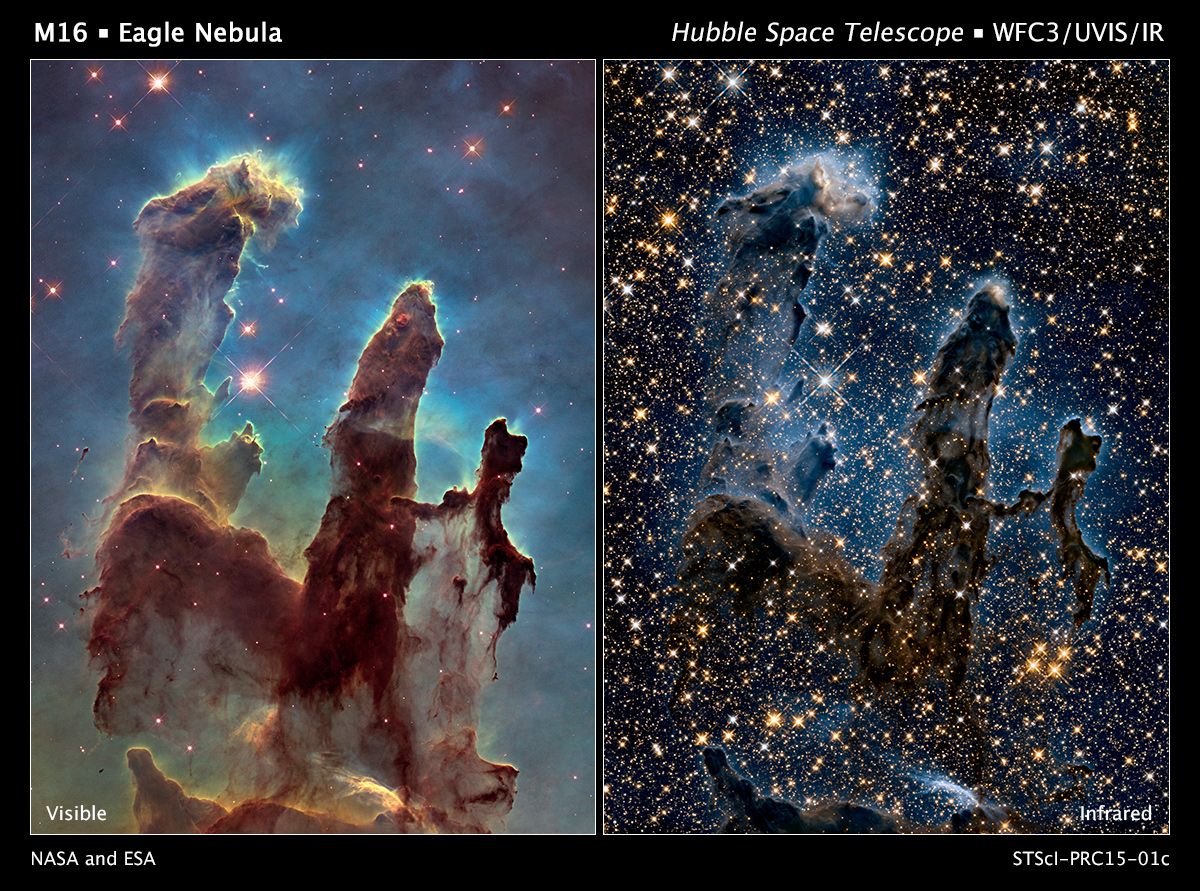
(Credit: NASA, ESA, and the Hubble Heritage Team (STScI/AURA))
Around each of these systems — both the successful stars and the failed ones — a large amount of material from the surrounding nebula accrues in either a disk or a series of disks: we call these protoplanetary disks. As with most systems of large numbers of particles, they quickly develop instabilities, which give rise to the earliest bound clumps of matter: planetesimals. These planetesimals interact, collide, smash one another apart and/or get stuck together, and gravitationally tug on one another.
Over relatively longer periods of time, some clumps will emerge as “winners,” where they vacuum up all of the matter surrounding them, and others will emerge as losers, where they either:
- get ejected from the system
- get consumed by another clump
- get slingshotted into (one of) the central mass(es)
- get torn apart by a collision or a gravitational encounter
Over time, both the central mass and the energetic light from the surrounding stars will blow most of the protoplanetary material away. When all is said and done, we’ll have a large number of new systems.
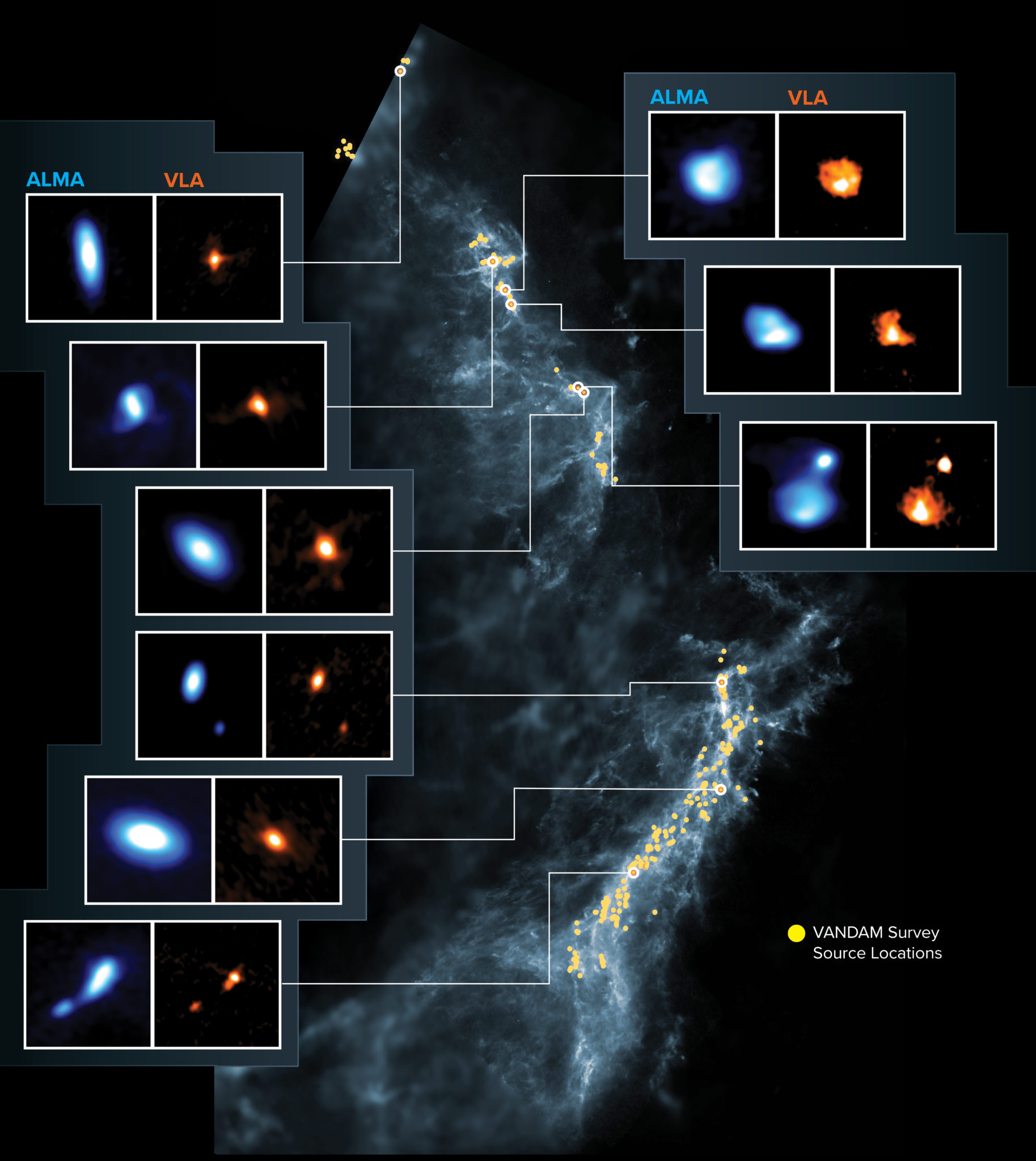
Credit: ALMA (ESO/NAOJ/NRAO), J. Tobin; NRAO/AUI/NSF, S. Dagnello; Herschel/ESA
What do these systems look like? A large number of them will have one or more stars in them, where you need to gather enough mass (about 8% of the Sun’s mass) to ignite nuclear fusion in the core. About half of the star-containing systems are like ours, with a single star and numerous planets, while about half have multiple stellar members in them, also with — as far as we can tell — planetary systems that orbit one or more stars.
The non-stellar objects that exist in these systems can be like Jupiter: massive and volatile-rich, and exhibiting self compression. They can be a little less massive: still rich in volatile gases, but without self-compression, like Neptune. Or they can not have volatiles at all, in which case they’re terrestrial, like Earth.
For every star that forms, there are multiple “failed stars” that also form, each of which can possess their own orbiting, smaller masses as well. This includes brown dwarfs and their systems, L and T Tauri “stars,” and what we could rightly call “orphan planets,” or masses that came into existence without ever having had parent stars at all.
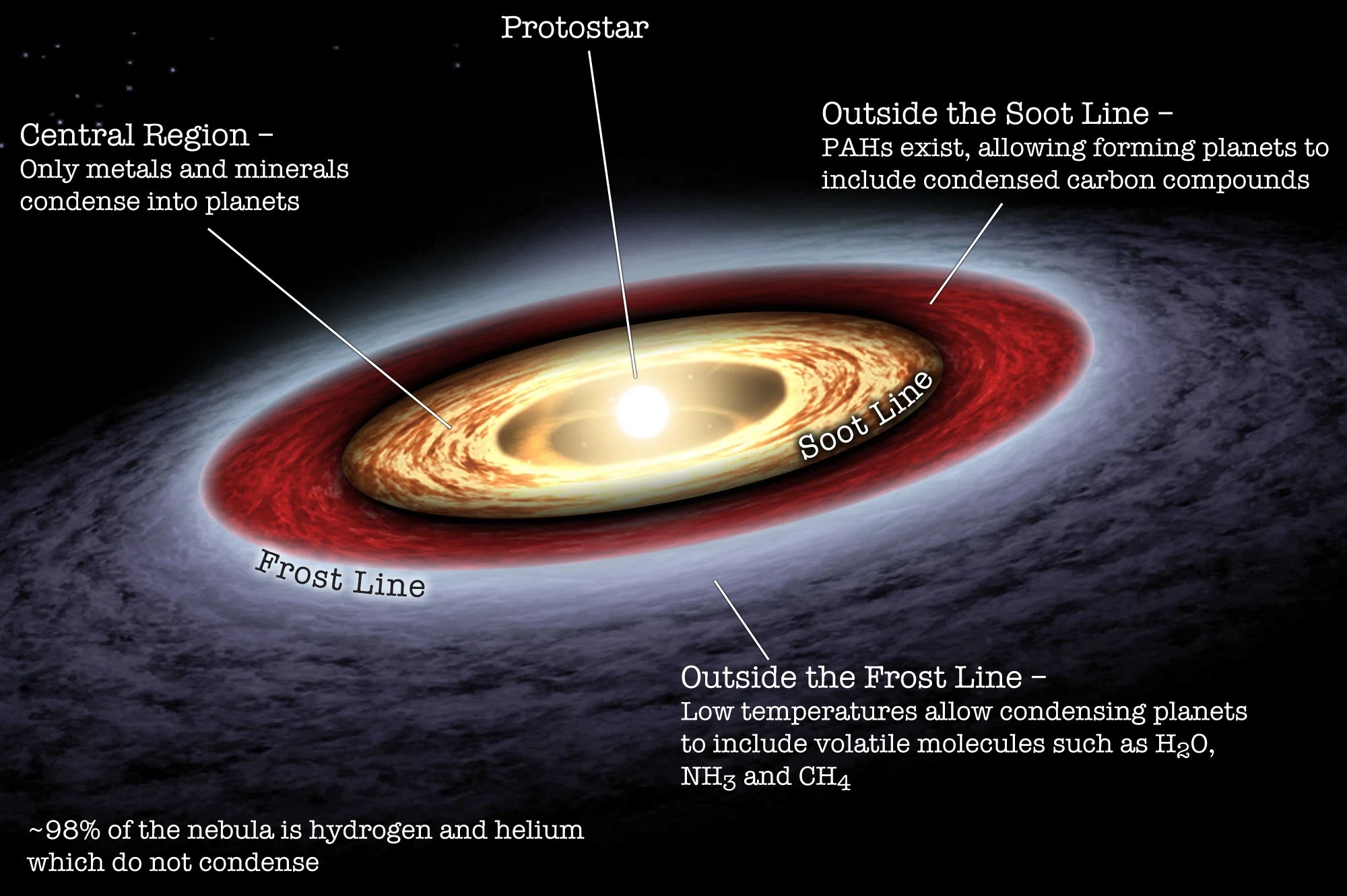
Credit: NASA/JPL-Caltech/Invader Xan
If we look only at the systems that contain at least one full-fledged star within them, we find that there are three separate “lines” that exist in each and every system.
- The Soot line. The innermost region of any solar system, closest to the parent star, will be extremely hot and subject to large amounts of radiation. No matter how massive you are, you cannot hold onto any volatiles; they’ll all be boiled away. Interior to the Soot line, only exposed planetary cores can exist.
- The Frost line. Back when a solar system’s planets formed, there was a line: interior to it, water-ice would be sublimated away into the vapor phase, while exterior to it, you could form stable, solid ice. This line corresponds to where the asteroids are present in our solar system: bodies that are largely rocky but also contain ices.
- The Kuiper line. Okay, I’ll fess up: nobody calls it this. But out beyond the final large, massive body that forms — the last one to sweep out all the other objects that share its orbit — are a large number of mostly icy bodies of various masses. These objects are composed almost exclusively of various ices and volatiles, and in our solar system they include the Kuiper belt and, beyond that, the Oort cloud. They can be as massive as Neptune’s Triton or as small as dust-grain-sized objects.

Credit: ESO/L. Calçada; Exeter/Kraus et al.
There’s a little bit more to keep in mind, as well. When we look at newly forming solar systems — the ones that still have their protoplanetary disks around them — we see that there are gaps in those disks, and we recognize that those gaps correspond to newly forming, likely quite massive, planets.
We know that if you want your object to pull itself into hydrostatic equilibrium, so that its shape is governed by gravity and angular momentum, an “exposed core” object that forms within the Soot line has to be approximately 10 times as massive as an object that forms outside of “the Kuiper line” and is composed solely of volatiles.
We also know that an object of a specific mass will only clear its orbit if it’s close enough to its parent star. The Moon would have cleared our current orbit if we took the Earth away and left our Moon behind; it’s massive enough. But Mars and Mercury would cease to have done so if we moved them out to the location of Eris. Similarly, Ceres could have been a planet, but only if it would have orbited at ~5% or less of the Mercury-Sun distance. When it comes to looking at what these objects of different masses can do in relation to their environments, as well as their internal, physical properties, we ignore the fact of their location — including where they formed — at our own peril.
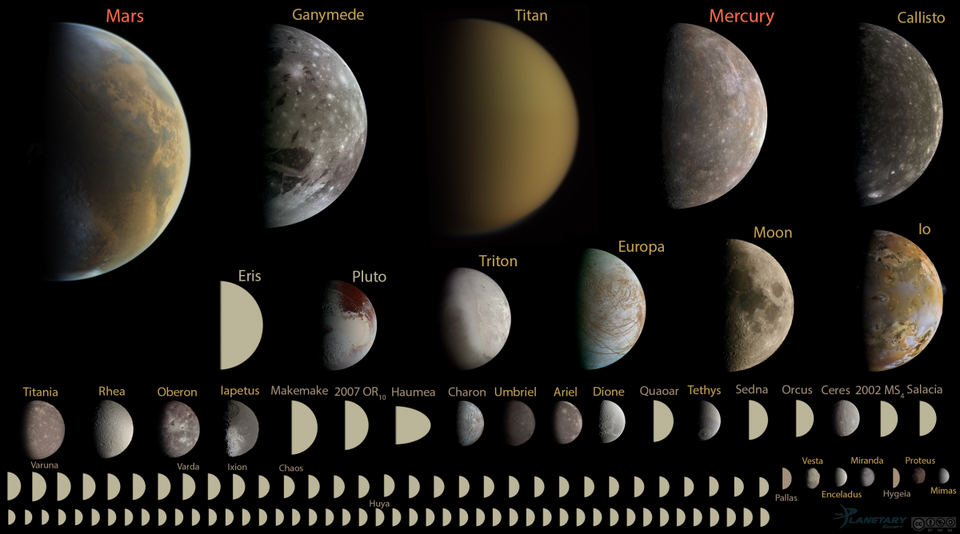
If we keep all of this in mind — the full diversity of the factors that lead to the formation of an object and the properties that it possesses — where is it useful to draw the dividing line between planet and non-planet?
Some, like Kirby Runyon, Phil Metzger, and Alan Stern, have advocated for what they call a “purely geophysical” definition: the characteristic of hydrostatic equilibrium alone determines your planethood. That’s one possible definition, but it ignores the wide variety of intrinsic and extrinsic properties that differentiate, say, Haumea from Mercury from Titan from Neptune. Each of those four worlds has the properties that it does because of where and how it formed, a fact we ignore at our own peril.
However, we can’t just use the International Astronomical Union’s definition, either. That definition has a terrible flaw in it: it only applies to objects that orbit the Sun, which means that every exoplanet around every other star in the Universe is not a planet. Fortunately, astrophysicist Jean-Luc Margot, back in 2015, extended the International Astronomical Union’s definition to planets outside of our solar system, even using a number of measurable proxies to accurately estimate what cannot be directly measured: whether an object has “cleared its orbit” or not.
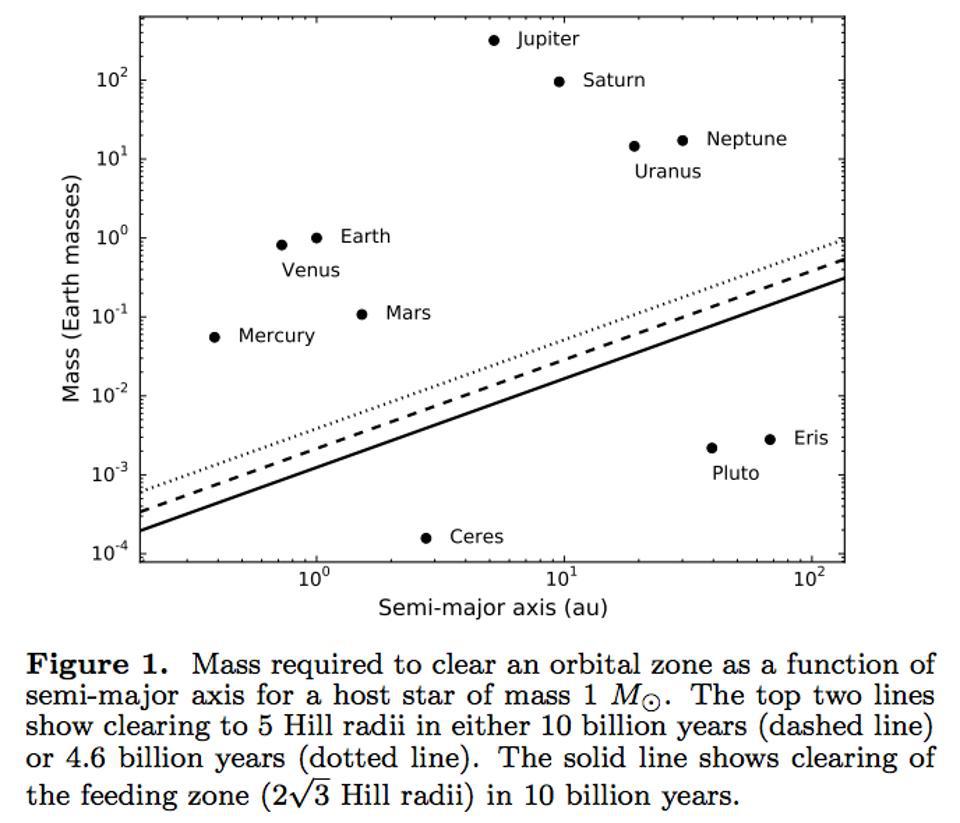
What’s probably more important than drawing another, different, equally arbitrary line between “planet” and “non-planet,” however, is to understand the different characteristics that objects with vastly different histories will possess.
- Objects that formed interior to the soot line will be denser and free of volatiles.
- Objects that formed between the soot and frost lines will be less dense, will have the capacity to possess volatiles, and can have a wide variety of masses.
- Objects between the frost and Kuiper lines will be less dense still, will be ice-and-volatile rich, and again can have a wide variety of masses.
- Objects beyond the Kuiper line will be made mostly of volatile ices, and all of those volatiles would likely boil away in short order if they are brought inside the frost line.
Meanwhile, objects ejected from a forming or fully-formed solar system will have different compositions and densities from objects that formed in a site that never possessed a parent star. Objects that formed from a circumplanetary disk, like Jupiter or Saturn’s large moons, are different than objects that migrate and get gravitationally captured, like Neptune’s large moon, Triton. When it comes to all objects less massive than stars, location and formation history — not simply mass and size — are vital factors in understanding what makes an object important or unimportant in any sort of scientific context.
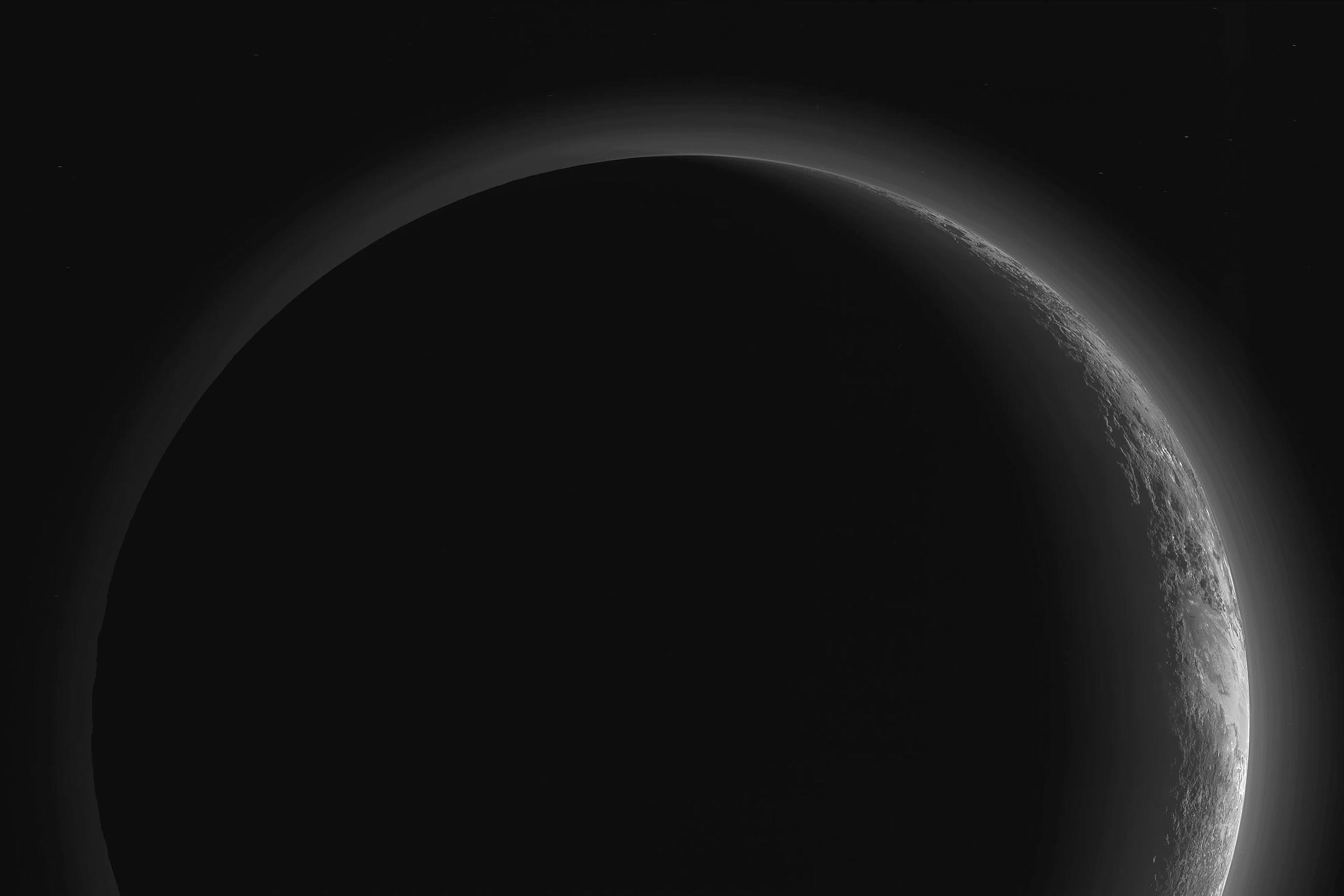
(Credit: NASA/JHUAPL/SwRI)
It’s always going to be unreasonable to demand that a classification scheme be universally applicable, and so there will always be dissenters and critics of any attempt to create one. However, it’s a far worse offense to water down a previously useful definition to the point of universal uselessness than it is to exclude a subset of one’s “favorite” objects from a designation that was previously assigned to them.
Still, based on what we can observe in the Universe, the fact remains that Pluto is completely unremarkable, as far as objects found beyond its solar system’s “Kuiper line” go. It has a perfectly normal mass, radius, composition, and formation history, and is a member of a population of objects that has very little in common with objects like terrestrial planets like Venus, ice giant planets like Neptune, and gas giant planets like Jupiter. There could be as many as ~1017 icy, round objects in the Milky Way galaxy alone, most of which aren’t bound to a parent star and never were. Unless one can make a compelling argument for why all of those objects should be classified as planets — despite how remarkably different they are from what we call a planet today — “Pluto as a planet,” based on the scientific merits, shouldn’t even be up for consideration.