Particle physics finally charts a healthy path forward
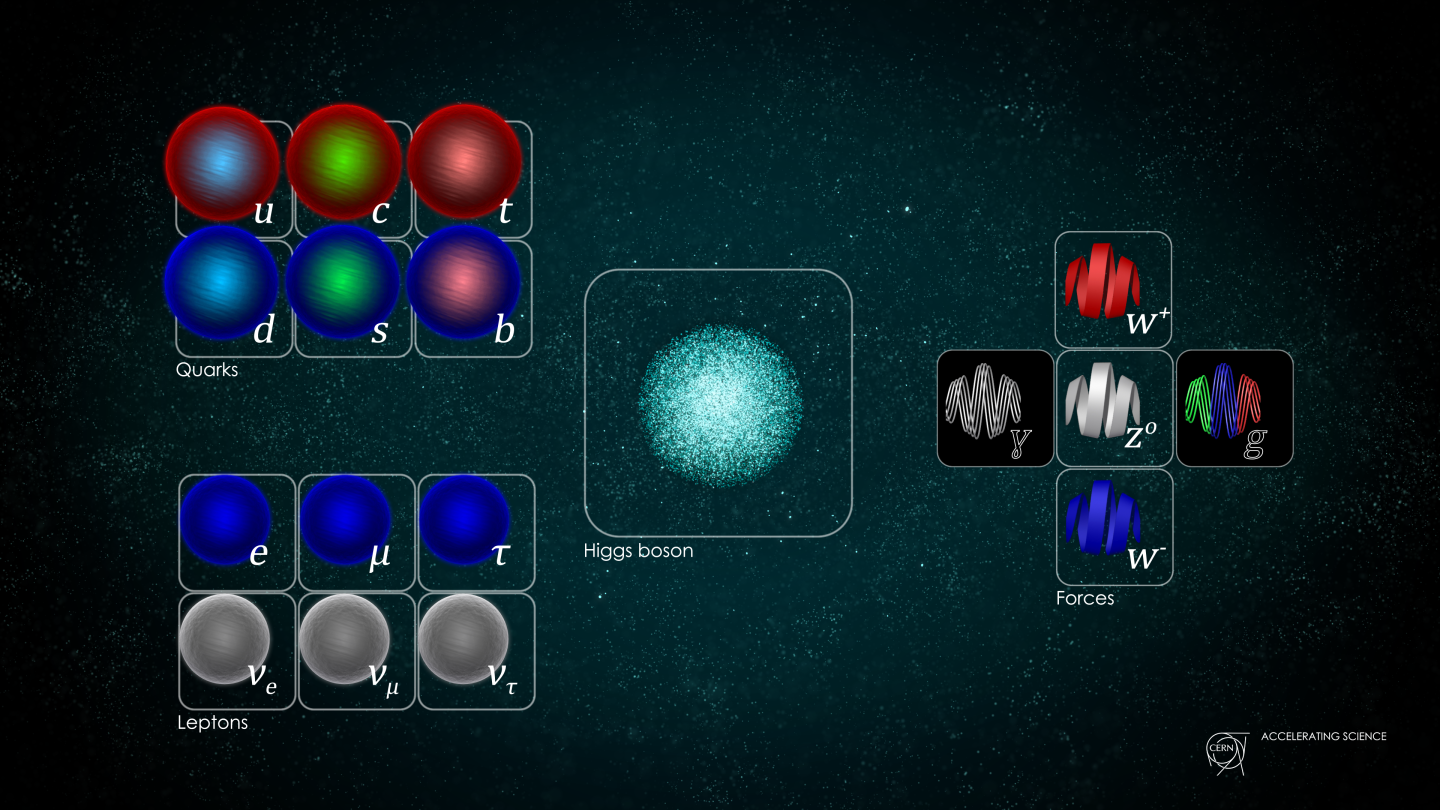
- The common perception of particle physics is that it's a completely wrongheaded endeavor: that we've known the Standard Model is true for ~50 years and just fail to go beyond it in progressively more expensive ways.
- The truth is very different: the Standard Model is only a framework, and we must both calculate and measure its properties exquisitely, especially if we wish to go beyond it someday.
- With the P5 report to chart the course for the next decade of particle physics, physicists show they finally get it: a broad and varied approach, with fewer assumptions, is how to create a healthy field.
Just a decade ago, the field of particle physics looked to be in a state of chaos. The Large Hadron Collider had recently turned on, and although they found the Higgs boson — the final undiscovered particle predicted by the Standard Model — it failed to turn up any evidence for any of the other leading theories that would take us beyond the Standard Model. Fermilab, the prior leader in the energy frontier, shut down its main accelerator permanently, and puzzles such as:
- the origin of neutrino mass,
- the nature of dark matter,
- and the origin of the matter-antimatter asymmetry,
seemed to be stagnating, with little progress to show on either the experimental or theoretical fronts.
Moreover, particle physicists themselves seemed to be bickering and in disarray as to what they should do next. Would there be enough value to justify a new, more powerful accelerator than the Large Hadron Collider? How should we be probing the behavior of neutrinos in order to understand neutrino mass? Are we taking sufficient advantage of the connection between particle physics and cosmology? Are we restricting ourselves to ill-motivated ideas in the hunt for dark matter? And are we devoting enough resources to supporting early career researchers and small-but-valuable experimental endeavors?
At last, with the release of the the Particle Physics Projects Prioritization Panel (P5) report, particle physicists have come together to chart a course for the next decade and beyond that addresses all of these issues while simultaneously creating a healthy environment for particle physics to thrive in the United States and the world for the next generation. Here’s what everyone should know.
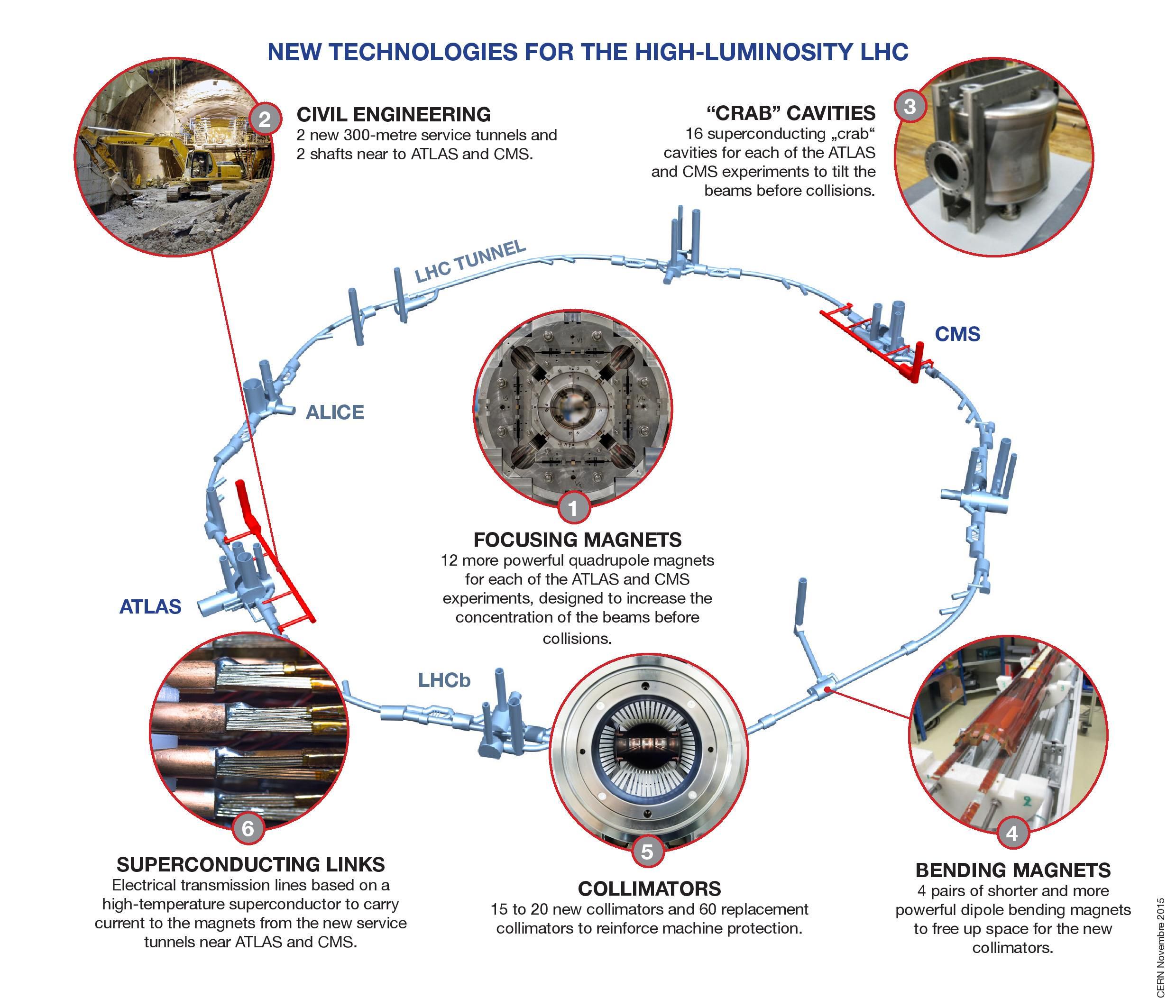
The flawed view of particle physics
There’s a common but oversimplified view of particle physics that, unfortunately, a lot of people seem to share. It goes something like this:
- The Standard Model has been known since the 1960s, and physics has stagnated ever since.
- Fundamental science is over, and theorists are only working on bad ideas that aren’t supported by reality.
- Experimentalists search for evidence of these bad ideas, only to find “nothing” to better and better precision.
- And that no meaningful progress has been made in particle physics in all the time since.
This view completely misunderstands what particle physics is, as well as how research is conducted.
The Standard Model isn’t actually a complete theory in the way that most people think: in the way that say Newtonian gravity, Maxwell’s electromagnetism, or Einstein’s general relativity is a theory. The Standard Model, rather, is a structural framework, which tells us that there are three generations of fermions, split into quarks and the charged and neutral leptons, with bosons that mediate the strong, weak, and electromagnetic forces. In order to know how the Standard Model manifests itself in our Universe, however, we need to make measurements. We need to find out things like the masses of the fundamental particles, the properties of mixing between quarks and the various species of neutrino, and what the types and amounts of violated symmetries are. Even knowing “the Standard Model is correct” only gets us partway toward understanding the properties of our Universe.

The truth about the Standard Model and beyond
We also know that the Standard Model is not all there is to reality. We know, for instance, that:
- the Universe was once more energetic, and probably much more energetic (up to 1011 times as much) as the highest-energy collisions achieved by the Large Hadron Collider,
- the Universe somehow is filled with both dark matter and dark energy, neither of which are accounted for by the Standard Model,
- the Universe somehow became filled with matter and not a corresponding amount of antimatter, even though all observed particle physics reactions show a matter-antimatter symmetry in that regard,
- and that many properties of our Universe, such as the masses of neutrinos or the low rest masses of fundamental particles compared to the Planck mass, are not explained by our best picture of reality.
We’ve been looking very hard at a number of well-motivated scenarios that have the theoretical potential to address these puzzles, and to date, experimental particle physics has placed enormously important constraints on these beyond-the-Standard-Model scenarios. We know that:
- the original version of supersymmetry (SUSY), which sought to solve the hierarchy problem,
- the original Peccei-Quinn symmetry, designed to solve the strong CP problem,
- and the Grand Unified Theory of SU(5), which sought to explain the matter-antimatter asymmetry,
are not the solutions to these puzzles that are borne out in our reality. Whatever the solutions are, they are more complex than these simplistic ideas.
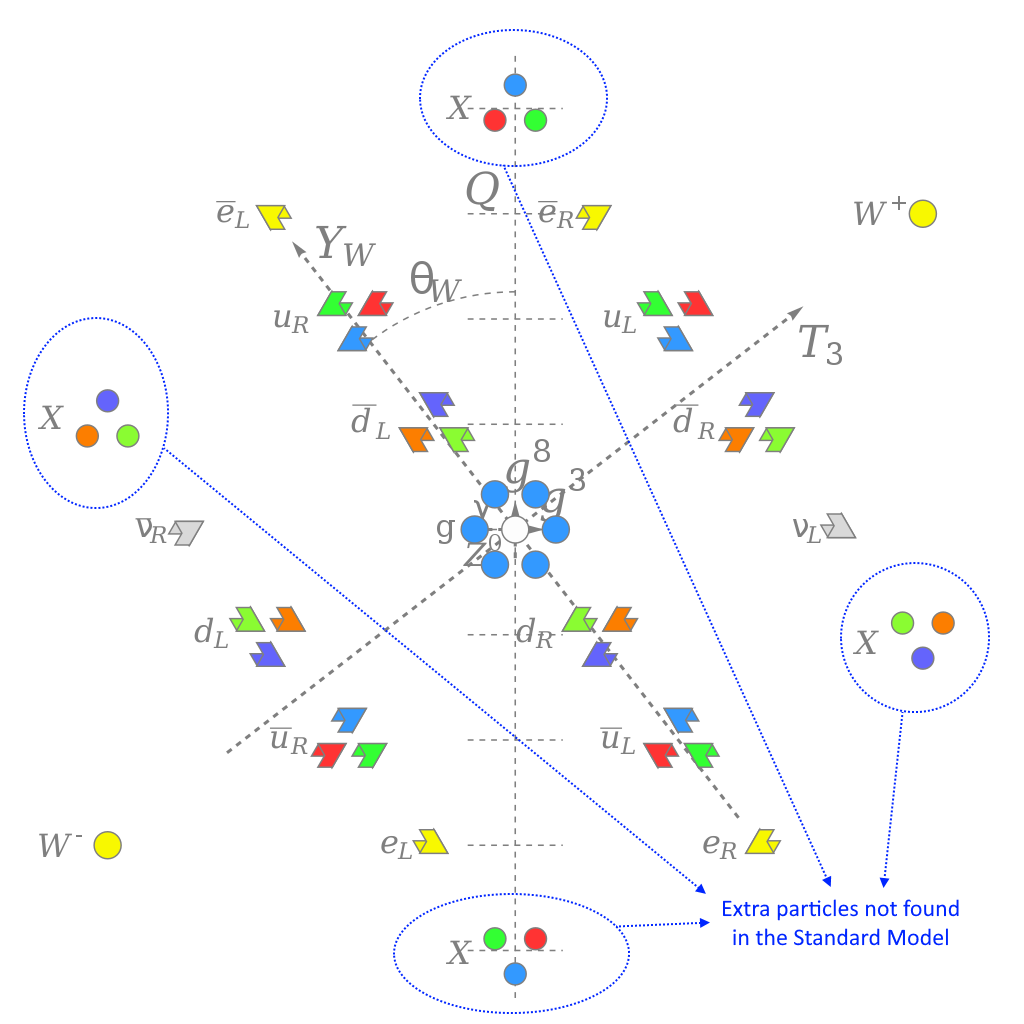
Investigating the greatest particle-based mysteries
However, these puzzles still persist, and are relevant to both particle physics and cosmology, as well as the intersection between the two. Remember: the hottest, densest, most energetic conditions in all of cosmic history were achieved in the first fraction-of-a-second after the start of the hot Big Bang. The earliest imprints of the inherent quantum nature of our Universe were seeded during cosmic inflation: a phase that preceded and set up the hot Big Bang that gave rise to us all. And that many of the puzzles we wonder about today, including:
- dark matter,
- dark energy,
- the nature of inflation,
- the origin of neutrino mass,
- and the physics of the Higgs boson,
very likely have their solutions imprinted in the earliest stages of our cosmic history.
In order to attempt to uncover the solutions to these mysteries, we have to take a variety of approaches. Yes, there are the brute force approaches of building high-energy colliders — with the greatest energies, detection sensitivities, and fastest rates of collisions possible — but there are also finesse approaches, such as searching for rare interactions and nuclear recoils, measuring the oscillations of neutrinos, performing cosmic observations that are sensitive to these ancient imprints, and developing new technologies and laboratory experiments to interrogate nature as never before. If we want to pin down exactly how nature operates, we have to ask the right questions in a clever enough way to compel it to give up its secrets.
That’s precisely what the P5 recommendations, at long last, get right.

High-impact investments within a sane budget
The goals of a scientific field like particle physics, at any point in time, are fourfold.
- To enable the science that’s going to address the big questions of the time, asking nature the key questions in ways that — if we are lucky — will lead to an increase in our understanding.
- To strike an appropriate balance between theory, which strives to understand how different scenarios would change phenomena that we can observe and measure, and experiment, which makes those measurements.
- To pave a roadmap for future research and support future researchers.
- And to do it all in a way that serves various different aspects of the community: from large research collaborations to small experiments to technological upgrades and innovations and more.
While the P5 report does strike a solid balance between these four competing priorities, the aspects that will be most exciting to most people are the major science efforts that have been designated as the highest priorities.

At the intersection of particle physics and cosmology are the quantum imprints left on the largest cosmic scales by the final moments of cosmic inflation. While we’ve detected, measured, and are working on understanding the density and temperature fluctuations (also known as scalar modes) that inflation left behind, there’s another type of fluctuations that inflation produces: gravitational wave fluctuations, also known as tensor modes.
All inflationary models predict the same spectrum of gravitational waves, but different models predict different amplitudes. The next-generation efforts of measuring the imprints (or lack of imprints) of these gravitational wave signals on the radiation left over from the Big Bang, known as CMB-S4 experiments, are one of the highest-priority science efforts recommended by the P5 report.
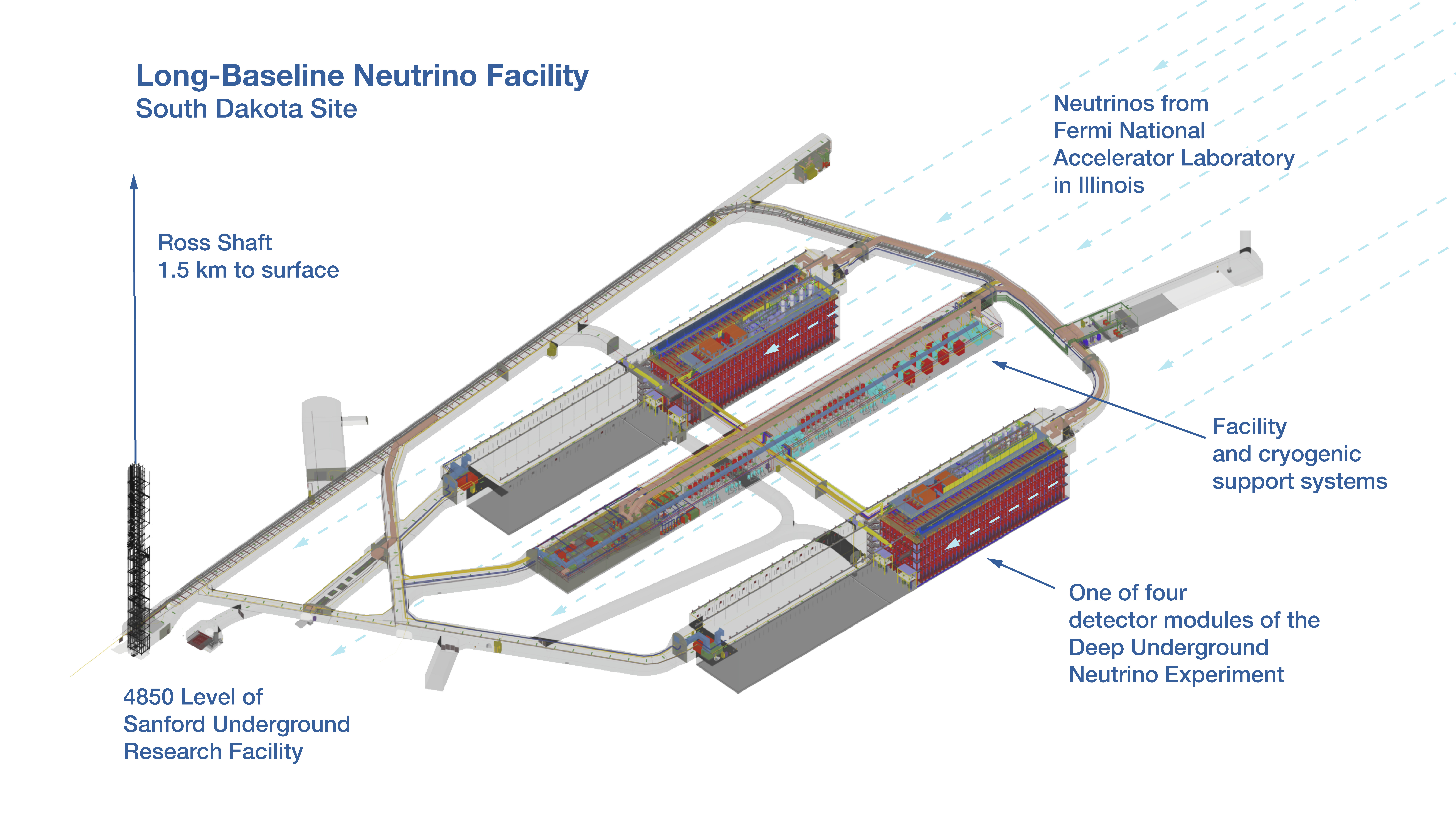
Perhaps the largest particle physics endeavor that’s taking place in the United States today is DUNE: the Deep Underground Neutrino Experiment. The goal of DUNE is to:
- generate a particle beam composed of an enormous number of unstable particles known as pions,
- to allow those pions to decay, where they’ll produce muons and muon neutrinos/antineutrinos (among other particles),
- to send these muon neutrinos (and antineutrinos) through the Earth, where they’ll have the opportunity to interact with the matter within the Earth,
- and then to measure the neutrinos, with a special type of detector known as a time-projection chamber, which arrive at a distant destination.
The reason scientists want to do this, and to do it with such large numbers of neutrinos to such high precision, is because neutrinos of one specific species — either electron, muon, or tau neutrinos — don’t remain that same species as they propagate, but oscillate between those various flavors. This kind of long-baseline neutrino experiment would represent a generational leap over all current efforts, and since the (massive) neutrino is the only known particle physics phenomenon that already goes beyond the Standard Model, it’s a puzzle that compels us to investigate it further. It deserves a high-priority investment.
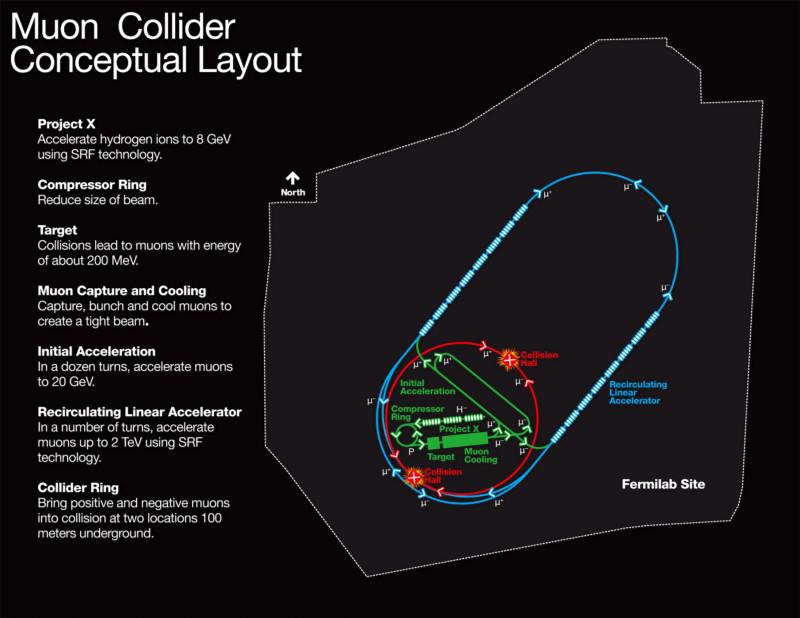
One super smart decision made in the P5 report is to hold off on investing deeply in any one of the proposed next-generation colliders, although the report recognizes the necessity of building a machine capable of acting like a “Higgs factory” to study this all-important Standard Model particle. Instead of shouldering that expense right now, they recommend supporting the technology development of various accelerator designs to see if there’s an advancement that makes it clear one design is superior to others. Right now, there are three main classes of accelerator design under consideration.
- A future circular collider, which would be a much larger ring than the Large Hadron Collider and which could collide either electron/positrons or hadrons (such as protons), leading to the highest energy collisions with the greatest new discovery potential.
- A future linear collider, which would collide electrons with positrons, leading to a very pristine signal but that does not push the energy frontier beyond current limits.
- Or a future circular muon collider, which would collide muons with antimuons, leading to high energies and pristine signals, but with the lowest number of total collisions due to the unstable nature of the muon itself.
While all of these options are expensive, a significant advance in energy gain-per-meter would strongly favor a linear collider, while an advance in muon luminosity would strongly favor the muon collider option: cleverly named a “muon shot” in homage to the Apollo program’s “moon shot” from the 1960s.
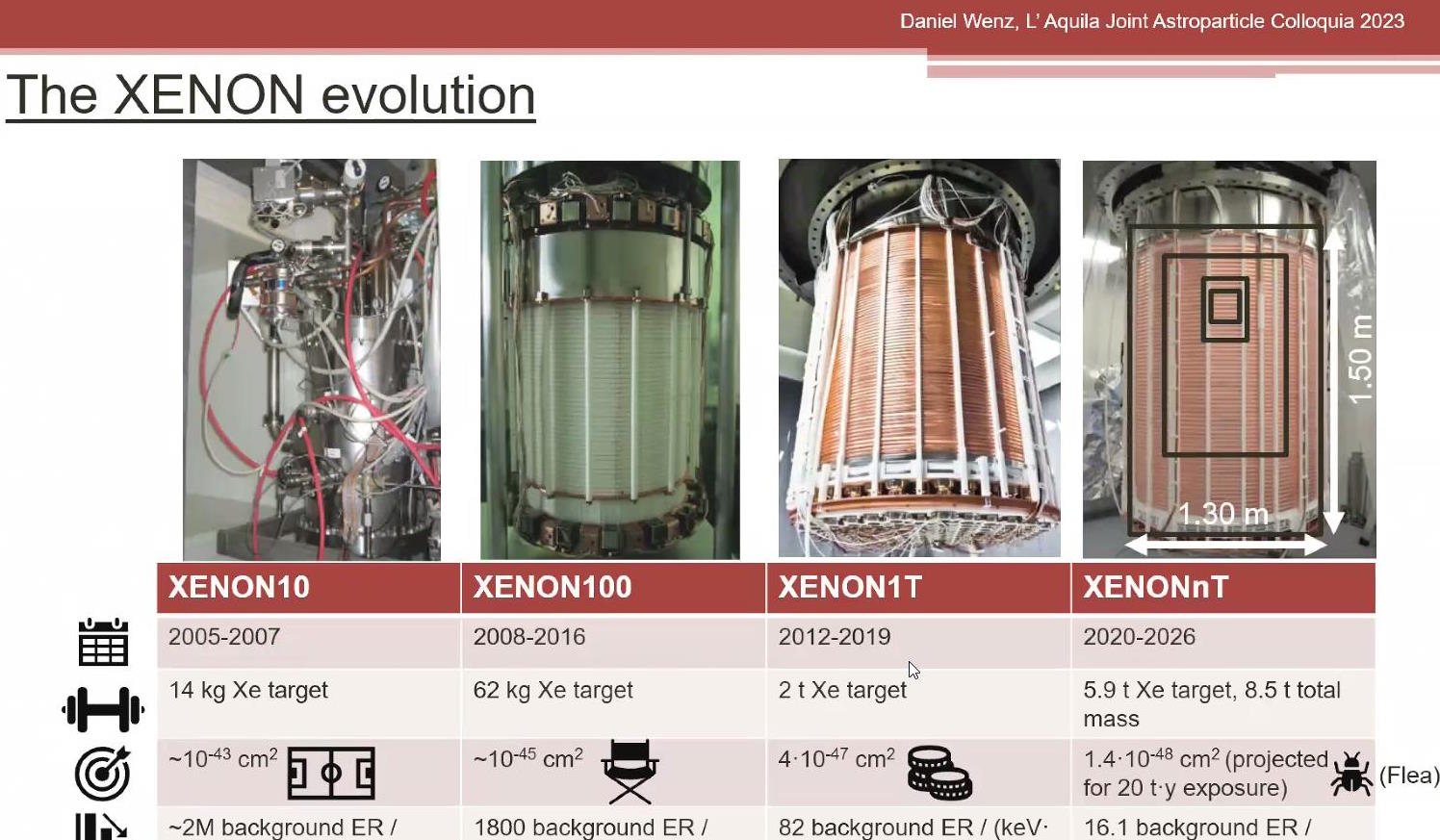
Also an incredibly high priority for particle physics is the construction of at least one “G3” (i.e., next-generation) dark matter direct detection experiment. A lot of people erroneously think that these dark matter experiments have been failures, but that’s simply not how science works. Science tells you where you ought to be looking, and experiments are the only way to find out what both is and isn’t there when you look. Over the past 30 years, our sensitivity to any exotic, massive particle that would interact with normal (atom-based) matter has increased by a factor of 320,000, signifying the best null result in all of science history.
The goal of these next-generation G3 experiments is to become so sensitive that they’re detecting — as part of their known background — what scientists call the “neutrino fog,” which is what cosmic neutrinos, neutrinos caused by radioactivity within the Earth, and neutrinos emitted by the Sun will induce inside the detector. Although these experiments were initially motivated by the search for WIMP-like dark matter, such as in supersymmetric or extra dimensional scenarios, it’s important to recognize that this approach is capable of detecting much more than just those favored models of dark matter, including other heavy, exotic dark matter candidates like Q-balls and WIMPzillas.
Particle physicists are recognizing the failed and arrogant approach of assuming we know what dark matter is going to be, and instead are casting a wider, broader, and more general net: evidence that they’re learning from the mistakes of prior generations.
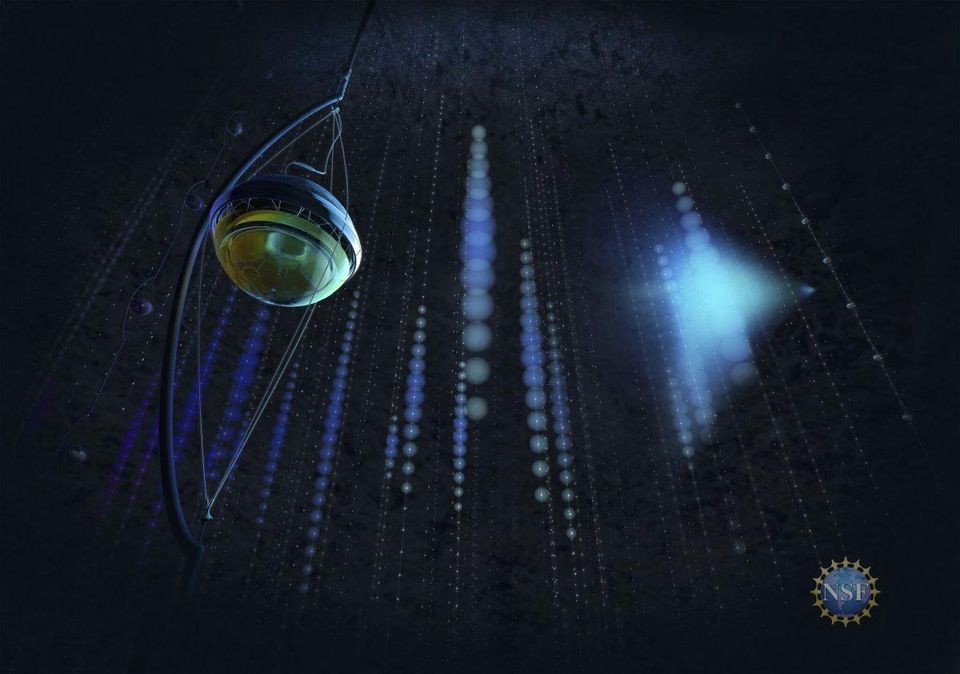
And as another important aspect of the future of particle physics, the community recognizes the vital importance of multi-messenger astronomy. Right now, there are three unique types of signals that can arrive from sources throughout the cosmos:
- light, in all of its forms, from gamma-rays to visible light to infrared all the way to radio waves,
- gravitational waves, now detected by not just LIGO and Virgo but also through pulsar timing,
- and cosmic particles, including in the form of neutrinos that can come from Solar System, galactic, and even extragalactic sources.
While the first two types of signals are mostly confined to the realm of astronomy, the ability to detect cosmic particles is of supreme interest to the particle physics community.
At the South Pole lies the IceCube detector: the best in the world at detecting cosmic neutrinos that interact with the icy matter around it. IceCube has already produced our first galactic map in neutrinos and has found neutrinos from an extragalactic source: a blazar, or an active supermassive black hole whose jet is pointed directly at us. Upgrading IceCube to the next generation of scope and sensitivity will enable unprecedented study of neutrino properties, and will enable the greatest multi-messenger astronomy program in history. As a bonus, if a supernova goes off within the Local Group, the next-generation IceCube facility will yield untold scientific riches.
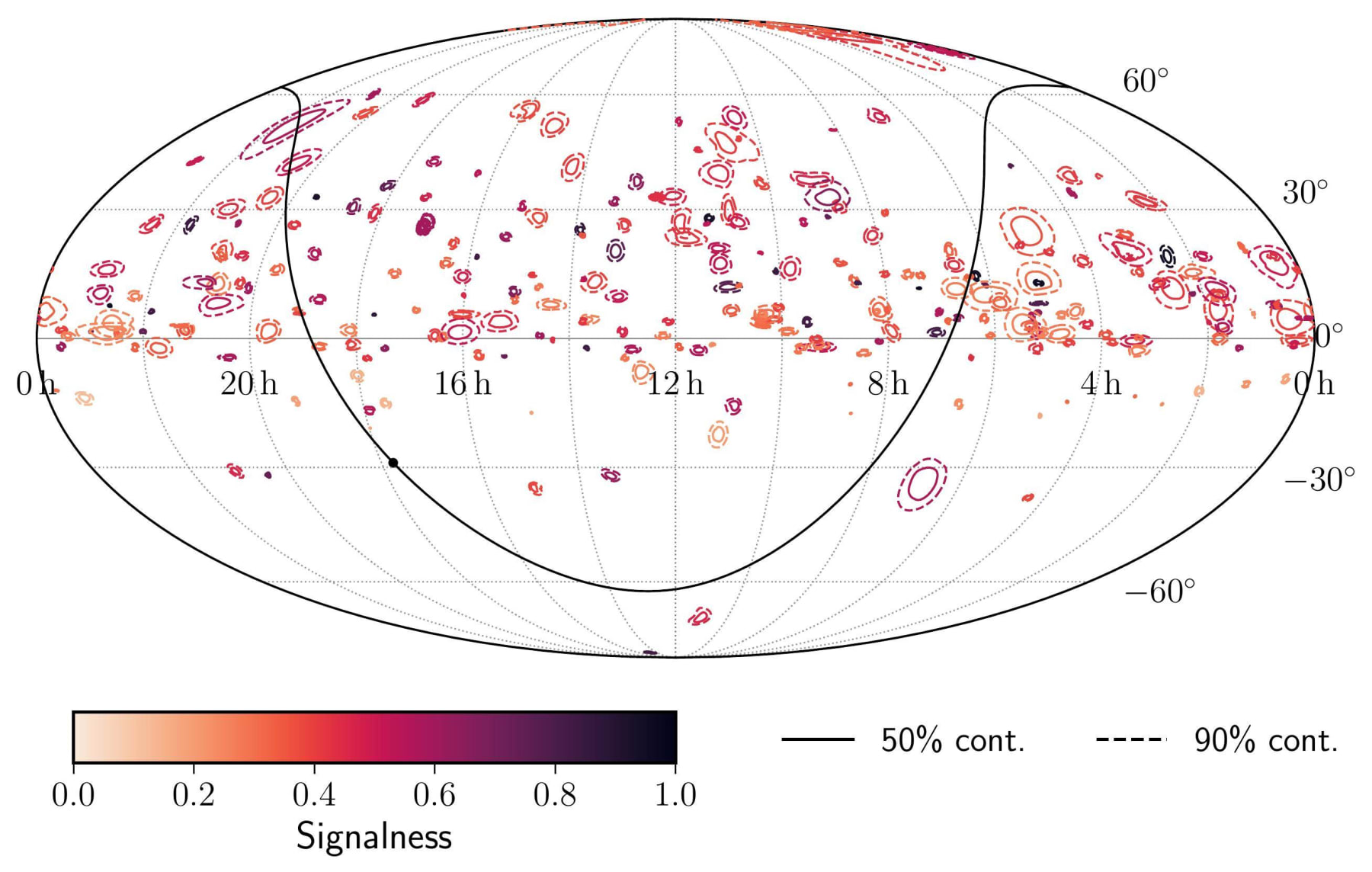
Conclusion & Summary
All of this comes along as part of a balanced portfolio within a sane, sober budget. Particle physicists are not asking for a tenfold or even a twofold increase in funding, but rather are keeping to an annual funding level from the US Department of Energy that’s between just $1B and $2B per year for the next decade. They are including support for early career scientists and many varied small experiments and projects: not just at national laboratories but also at universities and research centers. And they’re neither proposing a giant wish-list of every project imaginable nor demanding one ultra-expensive flagship project, but rather are trying to make targeted investments across a variety of efforts that give the physics community (and the general public) the greatest science “bang” for their buck.
It cannot be overstated how important an investment in basic, fundamental research is for the scientific future of any society. This kind of investment always, over the long-term, leads to greater economic activity and technological development, as well as scientific leadership in the world: the return-on-investment is staggering. But most importantly, the P5 report shows that particle physicists are finally learning from their past mistakes, and aren’t arrogantly overpromising discoveries about what might be out there beyond the frontiers of the presently known. There’s a Universe out there waiting for us to explore it. With the path laid out in the P5 report, particle physics just might have a bright future in the United States after all.