Ask Ethan: How did we prove the Big Bang took place?
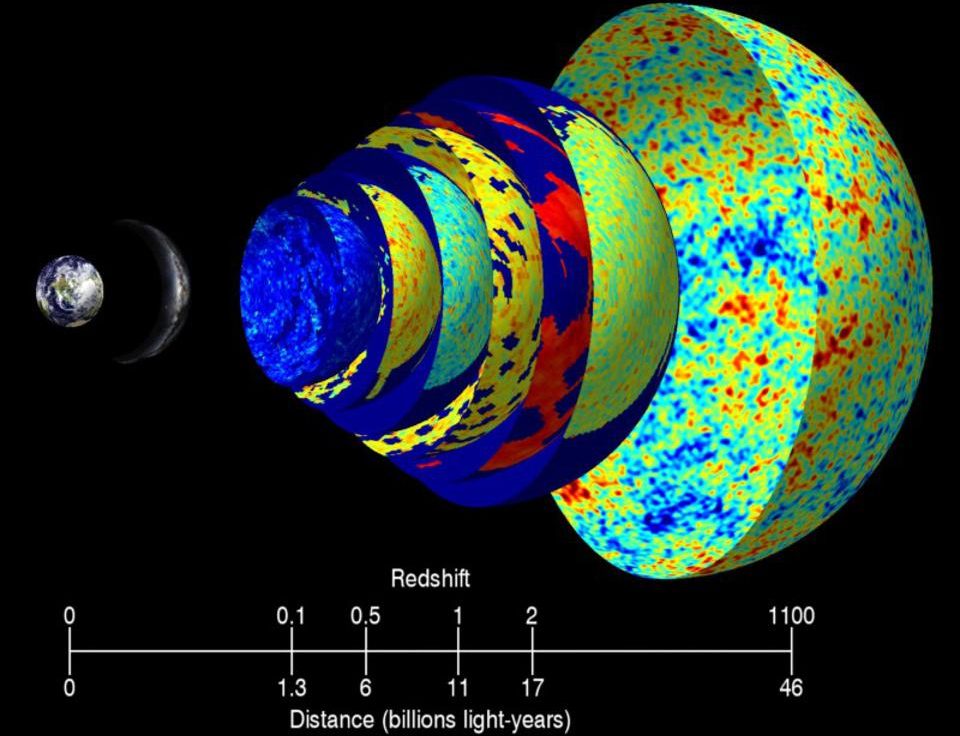
- One of the greatest discoveries of 20th century science was that the Universe as we know it hasn’t been around forever, but rather had an origin: the hot Big Bang.
- Although we take it for granted today, the Big Bang theory, when it was first proposed, was greatly debated and even derided by advocates of other, competing theories.
- Yet the decisive evidence that points to the Big Bang as a key event in the origin of our cosmos is unambiguous, and has withstood decades of challenges and scrutiny. Here’s how we know the Big Bang really happened.
Of all the great mysteries out there in the Universe, perhaps the greatest one of all is the question of our cosmic origin, “Where did all this come from?” For countless millennia, we told one another stories: of a fiery birth, of the separation of light from dark, of order emerging from chaos, of a dark, empty, formless state from which we emerged, or even of an existence that was eternal and unchanging. Some stories involved an active creator; others needed no intervention from anything other than nature itself. But despite our propensity to believe in one of these stories or another, in science, we don’t settle for belief: we want to know.
Today, we talk about the Big Bang as though it’s foundational and taken for granted. But that wasn’t always the case. So how did we get to this point? What critical scientific steps occurred to promote the Big Bang from just one among many ideas to a scientific certainty? That’s what Muhammed Ayatullah wants to know, as he writes in and asks, simply and straightforwardly:
“How was it proven that the Big Bang actually took place?”
It’s a story that started long before it was proven. Let’s go back to when the idea was first conceived: nearly a full 100 years ago.

Back in 1915, Einstein shook up our understanding of the Universe by publishing his theory of General Relativity: a radically new conception of gravity. Previously, Newton’s law of universal gravitation was how we conceived of gravity, where space and time were absolute quantities, that masses occupied certain positions in space at certain moments in time, and that every mass exerted a force on every other mass, inversely proportional to their distances. This explained most observed phenomena very well, but fell short under a few physical circumstances: at speeds that began to approach the speed of light, and in very strong gravitational fields, where you were only a short distance away from a large mass.
Einstein first did away with absolute space and absolute time, replacing them with a unified structure that wove the two together: the four dimensional fabric of spacetime.
Next, he had what he’d later refer to as his happiest thought: the equivalence principle. He recognized that if an observer, like a human being, were in a closed room, and that room were accelerated upward by some sort of engine, you’d feel a force pulling you down. He also recognized that if the room were stationary on the surface of a planet like Earth, you’d also feel a force pulling you down. In fact, if all you could see and measure was the inside of the room, you’d have no way of knowing whether you were accelerating or gravitating: your experience of the two very different physical situations, somehow, would be equivalent.

It was this realization that led him to formulate General Relativity, where gravitation was just another form of acceleration, and if your acceleration wasn’t due to an external force, then it must come from the Universe itself: due to the curvature of the fabric of spacetime. As John Wheeler would put it years later, matter and energy tell spacetime how to curve, and that curved spacetime, in turn, tells matter and energy how to move.
So what would happen if you had a large, enormous Universe that obeyed these gravitational laws — the rules of General Relativity — and you filled it, uniformly, with matter and/or other forms of energy?
According to Einstein’s theory, it couldn’t remain static in any stable sort of way. Spacetime doesn’t just curve and bend due to the presence of matter and energy, it can also evolve by either expanding or contracting. When you work through General Relativity’s equations for these conditions, that’s precisely what you find: the Universe must either be expanding or contracting. This was derived all the way back in 1922 by Soviet scientist Alexander Friedmann, and the equations that bear his name are still, in many senses, the most important equations in all of cosmology.

But it would be irresponsible to rely on theory, alone, for drawing any sorts of meaningful conclusions about the Universe. In science, we always demand experimental confirmation of any theory before we dare to accept it. In the science of astronomy and astrophysics, however, we don’t have the luxury of moving planets, stars, and galaxies around like we would in a laboratory setting. When it comes to experimenting on cosmic phenomena, we do it observationally: the Universe is our grand laboratory. All we have to do is observe the relevant systems doing the things we’re interested in, and that will uncover the best approximations of what’s true about reality.
The key observation was to look at the spiral and elliptical nebulae in the sky. Back in the 1910s, an astronomer named Vesto Slipher had begun to observe emission and absorption lines from these galaxies, and realized they must be moving very rapidly: some toward us, but most moving away from us. Then, starting in 1923, Edwin Hubble and his assistant, Milton Humason, began at long last measuring the other critical component to the equation: the distances to these nebulae. As it turned out, most of them were millions of light-years away, with some being even more distant. When he graphed out distance versus recession speed, there could be no doubt: the farther away a galaxy was, the faster it appeared to recede.

There were many interpretations of why this would be the case. Hypotheses included assertion that the Universe:
- violated the principle of relativity, and that the light we observed from distant objects simply got tired as it traveled through the Universe,
- was the same not only in all locations, but at all times: static and unchanging even as our cosmic history unfolded,
- didn’t obey General Relativity, but rather a modified version of it that included a scalar field,
- didn’t include ultra-distant objects, and that those were nearby interlopers that observational astronomers were confounding for distant ones,
- or that it began from a hot, dense state and had been expanding and cooling ever since.
However, if you put Friedmann’s theoretical work (within the context of General Relativity) together with Hubble’s, Humason’s, and Slipher’s observations, it became clear that the Universe was not just like a fabric, but that the fabric was expanding over time. The Universe was like a leavening ball of bread dough with raisins all throughout it: the raisins were like galaxies, and the dough was like spacetime. As the dough leavens, the raisins recede from one another: not because they’re moving through the dough, but because the dough itself is expanding.

The first person to put all of this together, though, wasn’t Hubble himself, even though we named the law that governs the expanding Universe (and the telescope whose goal was to measure the rate of that expansion) after him. Instead, it was a Belgian priest named Georges Lemaître who did it, way back in 1927: back when Hubble’s observations were still in their very early stages. He pointed to these observations as evidence for the expanding Universe, and extrapolated it backward in time: if the Universe is sparse and expanding today, then back in the distant past, it must have been denser, smaller, and more uniform, because it hadn’t had time to gravitate and clump up just yet.
In a fun twist of history, Lemaître sent his preliminary results to Einstein, who was aghast at them. In his response, Einstein wrote back to him, “Vos calculs sont corrects, mais votre physique est abominable,” which means “Your calculations are correct, but your physics is abominable!”
But even though as towering a figure as Einstein was derisive toward his conclusions, others soon caught on. In 1928, Howard Robertson, independently, drew the same conclusions. Later, Hubble himself came around, as did Einstein, eventually. But the next great advance would come in the 1940s, when George Gamow began expanding on these ideas.
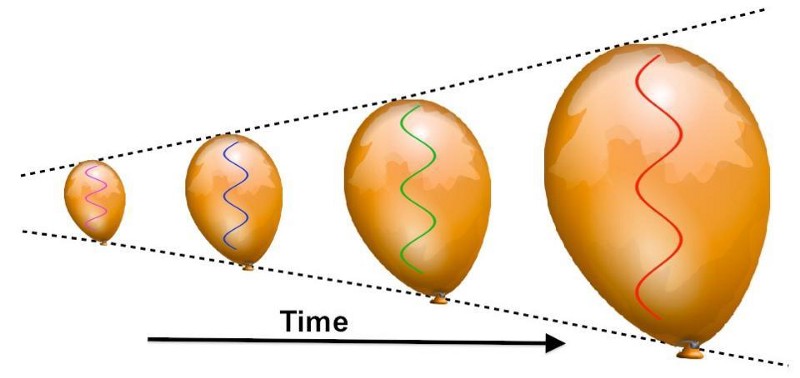
Gamow was actually a student of Alexander Friedmann back in the early days of his studies, before Friedmann’s untimely death in 1925. As he began studying astrophysics, Gamow became enamored of Lemaître’s ideas and extrapolated them still further. He realized that if the Universe was expanding today, then the wavelength of the light that travels through the Universe must increase over time, and therefore the Universe was cooling. If it’s cooling today, then if we were to run the clock of the Universe backward instead of forward, we’d discover a Universe with light of shorter wavelengths. Because energy and temperature are inversely proportional to wavelength (short wavelengths are higher in temperature and energy), the Universe, therefore, must have been hotter in the past.
Extrapolating backward, he recognized that there must have once been a time period when it was too hot for neutral atoms to form, and then a period before that when it was too hot for even atomic nuclei to form. Therefore, as the Universe expanded and cooled from an early, hot, dense state, it must have formed the first stable elements and then, later, neutral atoms for the first time. Because photons couple tightly to free electrons but not to neutral, stable atoms, this should result in the existence of a “primeval fireball,” or a cosmic background of cold radiation, created from this early plasma. Given the billions upon billions of years that must have passed for cosmic evolution to give rise to the Universe as we see it today, that radiation background should only be a few degrees above absolute zero by the present.

For many years, there were intense theoretical arguments about the Universe’s origins, but no decisive evidence. Then, in the 1960s, a team of physicists at Princeton, led by Bob Dicke and Jim Peebles, began to calculate the explicit properties that this leftover background of radiation should have.
Back in the early stages of the Universe, photons would exist amidst a sea of ionized plasma particles: atomic nuclei and electrons. They would collide with these particles constantly, particularly the electrons, thermalizing in the process: where the massive particles achieve a particular energy distribution that’s simply the quantum analogue of a Maxwell-Boltzmann distribution, and the photons wind up with a particular energy spectrum known as a blackbody spectrum.
Once neutral atoms form, the photons simply travel throughout the Universe in a straight line, and will continue to do so until they run into something that absorbs them. But because they exist within the expanding Universe, they should redshift, cooling to very low temperatures by the present. They planned to build a radiometer and fly it up to high altitudes, where they hoped to observe this leftover glow of radiation.

But just 30 miles away, in Holmdel, New Jersey, a story would unfold that would render this experiment moot before it ever launched. Two young scientists, Arno Penzias and Bob Wilson, were put in charge of a new instrument: the Holmdel Horn Antenna at Bell Labs. Originally designed for radar work, Penzias and Wilson were attempting to calibrate their instrument when they noticed something funny. No matter where they pointed the antenna, the same amount of “noise” appeared everywhere. They tried everything:
- recalibrating it,
- shutting all the systems down and restarting them,
- even going into the horn itself with mops and removing all the bird’s nests and droppings inside.
But nothing worked; the noise remained. It didn’t exist if it was pointed at the ground, and it only varied if it was pointed at the plane of the Milky Way or the Sun itself.
Finally, a scientist who just happened to be refereeing one of Peebles’s papers came to Holmdel, when Penzias and Wilson told him of their woes. He tipped them off, and they called up Bob Dicke at Princeton. After a few minutes on the phone, Dicke’s voice rang out through the halls, “Boys, we’ve been scooped!” The leftover glow from the Big Bang had just been discovered.

Or had it?
Today, we know this to be the case, but many alternative explanations were initially put forth. Perhaps this wasn’t the leftover glow from the Big Bang: a primeval fireball. Instead, perhaps it was some sort of reflected starlight, which had heated up cosmic dust in all directions, which was then re-radiated back in all directions, where the antenna picked it up. Since stars are ubiquitous and dust is ubiquitous, perhaps these two effects could combine to create a similar leftover glow, again, just a few degrees above absolute zero.
The way to differentiate between the two isn’t just to discover the presence of this radiation background, but to measure its spectrum: how its intensity varies with frequency. Remember, the prediction from the Big Bang is that this would be a perfect blackbody spectrum, and that the photons left over from the Big Bang would follow that perfect temperature distribution predicted by a body at a single temperature in thermal equilibrium.
But starlight isn’t quite like that. Our own Sun, for example, isn’t well-represented by a single “body” radiating at a single temperature, but by a series of blackbodies superimposed atop one another, corresponding to the different temperatures present in the outermost few hundred kilometers of the Sun’s photosphere. Instead of a blackbody spectrum, the light should be represented by a smeared-out distribution that was quantifiably different.

And those two scenarios are something that more modern experiments — throughout the 1970s, 1980s, and culminating with the COBE observations (from space) in the 1990s — definitively established. It wasn’t through dogma or wishful thinking or assuming the conclusion and then working backward that the Big Bang was established; it was because there were explicit predictions that the Big Bang made that were different from the predictions of every other theory, and when we took the critical observations, the Big Bang was the only survivor: the only one that agreed with the full suite of what was seen and measured.
In science, that’s as close as we get to a proof. Science, remember, is not mathematics; you cannot formally “prove” that something is a certain way. What you can do is establish that one particular set of ideas is valid: consistent with everything observed and measured within the Universe, and show how that stands in contrast to other, competing ideas that fail to agree with the observations and measurements that have been taken. That’s how we established the Big Bang as our best model of where our Universe comes from, and why, even though we now use the Big Bang as our foundation to build further atop it, it remains undisputed as an early, hot, dense, expanding state as part of our cosmic origin story.
Send in your Ask Ethan questions to startswithabang at gmail dot com!