The Big Bang: a series of steps down an energy staircase
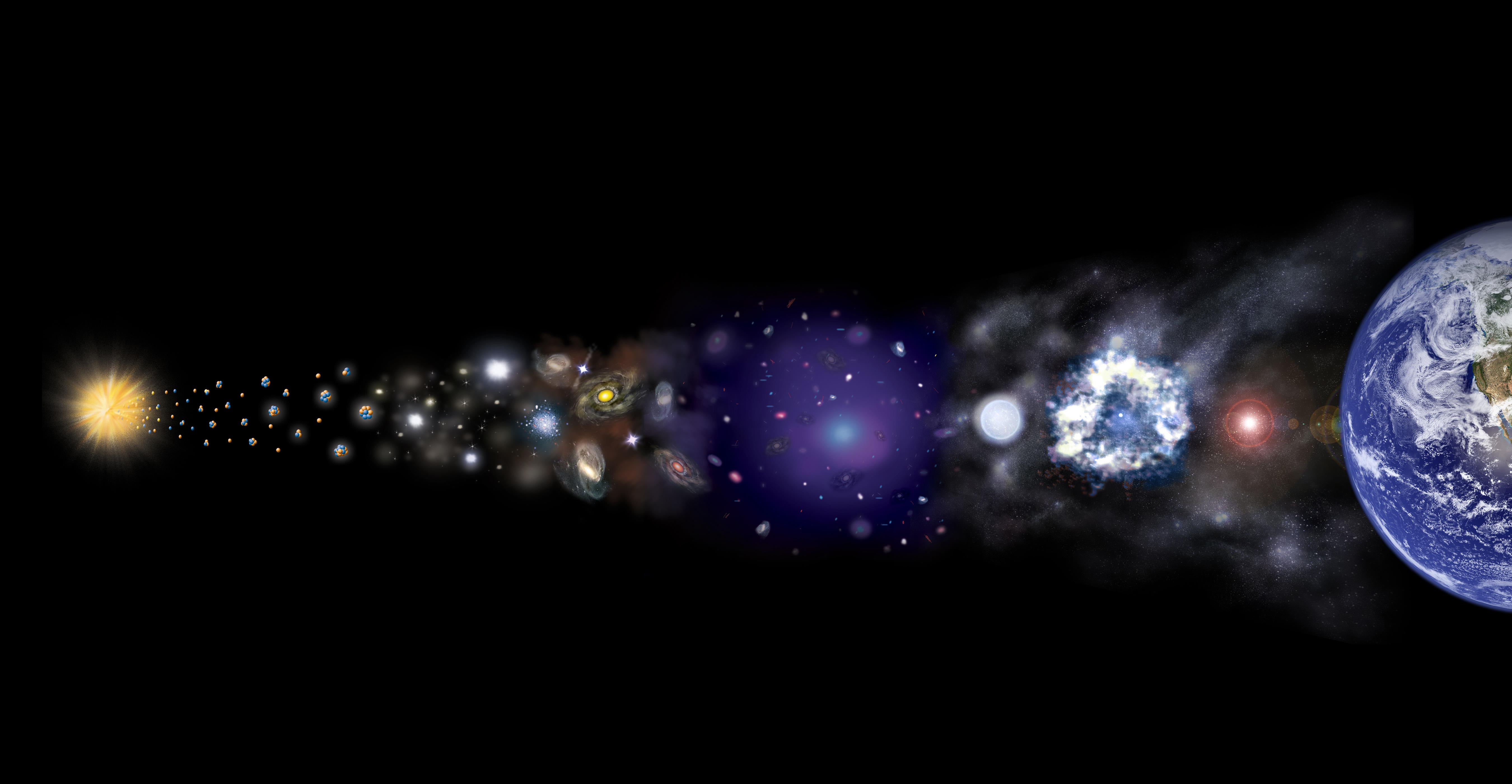
- At the start of the hot Big Bang, the Universe had almost unlimited potential: with a tremendous amount of energy inherent to an enormous number of fast-moving quanta of a variety of species.
- As time elapsed, however, the Universe expanded and cooled, with all of the quanta within the cosmos not only suffering the consequences, but stepping down in energy and increasing the total entropy of the Universe.
- What we wind up with, in the aftermath of this early, violent history, is a Universe ready to form atoms, molecules, stars, and later on, rocky planets and life. Here’s how it all unfolds.
Try, if you dare, to imagine what the Universe was like right at the start of the hot Big Bang. Everywhere, all at once, space was populated by a primordial bath of quanta: particles, antiparticles, photons, gluons, and more, including anything and everything whose existence is possible with enough energy. In each moment that elapses, particles collide in enormous numbers — sometimes annihilating, sometimes transmuting from one species to another, sometimes creating new quanta via Einstein’s E = mc² — but the Universe also expands. As the Universe expands, it cools, with each quantum losing energy, having its wavelength stretched, and causing the density of the Universe to drop, which further decreases the overall collision rate.
Eventually, after hundreds of thousands of years pass, neutral atoms stably form. Millions of years later, a sufficient amount of gravitational collapse has occurred that enormous, massive clouds composed of those atoms begin to form stars for the first time, giving rise to both the seeds of modern-day galaxies and the heavy elements that would enable the formation of rocky planets and, ultimately, life.
However, it was a long journey during the first few minutes of cosmic history to get from a hot, dense, uniform state to a cool one poised to form neutral atoms. The story of the early Universe, from the Big Bang until neutral atoms would stably exist, is the story of a series of steps down an energy staircase. Here’s what must have unfolded.

The onset of the hot Big Bang is defined by the earliest moments that our Universe was filled with energetic quanta: all the known particles and antiparticles of the Standard Model, plus (likely) many others that we have yet to identify, in a highly symmetric state. The quantum rules that govern our Universe — at least, as we understand them — tell us that there were only three fundamental forces at this time: the strong nuclear force, the electroweak force, and gravitation. They also tell us that although each of the quanta of the Standard Model possess properties like electric charge, color charge, and an inherent energy, they do not yet possess a non-zero rest mass, and that for any fermion (particles with spin-½, as opposed to integer spins) that gets spontaneously created via E = mc², its antiparticle counterpart must also be created in equal numbers.
There is definitely some unknown physics that happens in these early stages of cosmic history. Some species of dark matter, presently unknown to humanity, must have been created: something that cannot be a part of our Standard Model. Some sort of process must have occurred to create a fundamental asymmetry between matter and antimatter: where more fermions (specifically, quarks and charged leptons) than antifermions (antiquarks and charged antileptons) were created. And, potentially, some other set of exotic physics processes that exists only in the realm of the speculative at present may have occurred as well. The first femtosecond or so (~10-15 seconds) of the hot Big Bang, other than the facts of cosmic expanding and cooling, remains largely unexplored by modern physics and astrophysics.
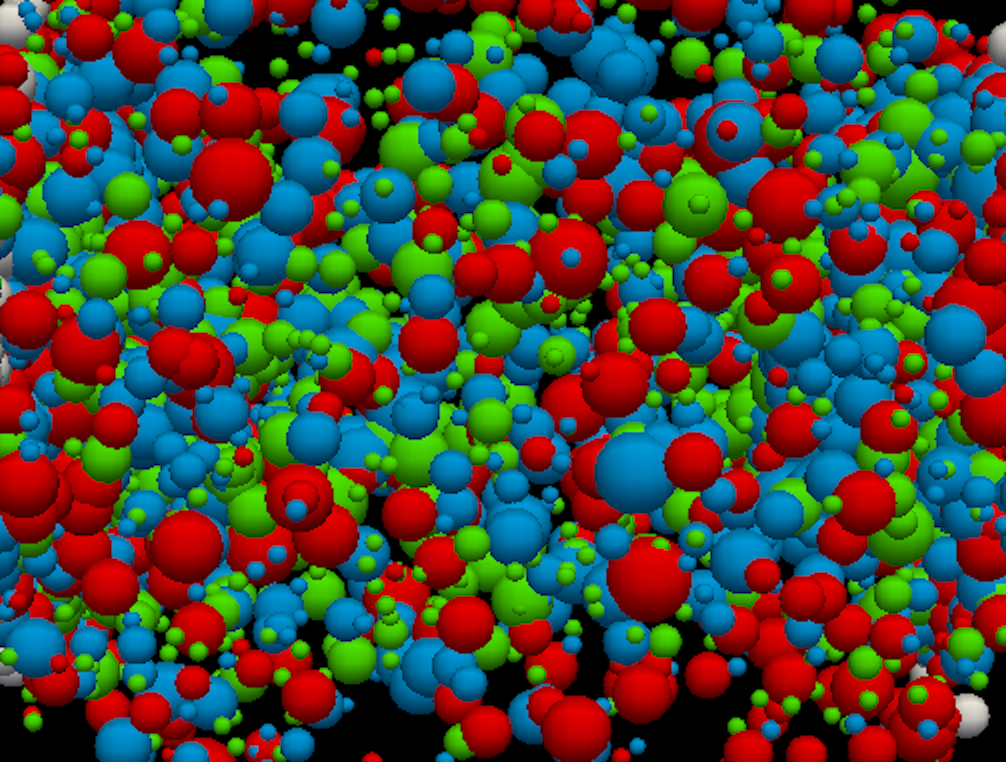
Once the first ~10-13 seconds or so have elapsed, however, things begin to change from the hot, dense, uniform state that started off the hot Big Bang. Conditions are now about a factor of a trillion (1012) cooler than they were at the hot Big Bang’s onset, with temperatures “only” of a few quadrillion K at the moment. That’s cool enough so that it becomes quite difficult to create the most energy-demanding quanta, which also happen to be the shortest-lived (i.e., most unstable) particles and antiparticles in the Standard Model. In particular, it becomes harder to create them (via E = mc²) than it is to destroy them. Top quarks and antiquarks begin to disappear from this primordial particle-antiparticle soup, decaying away faster than the Universe can recreate them.
While something similar may inevitably be in store for most of the other Standard Model particles in due time, something else happens as the Universe approaches a picosecond (10-12 seconds) in age: the electroweak symmetry breaks. This coincides with a number of important transitions.
- The electroweak force splits into the electromagnetic force and the weak nuclear force; electroweak unification has ended.
- The Higgs symmetry breaks, causing the Higgs field to “stiffen,” which gives the particles and antiparticles of the Standard Model their rest masses.
- And the W-and-Z bosons also gain masses as they “consume” some of the degrees-of-freedom arising from these broken symmetries.
In the aftermath of these transitions, the particles and antiparticles of the Standard Model now have rest masses, and experience up to four fundamental forces.
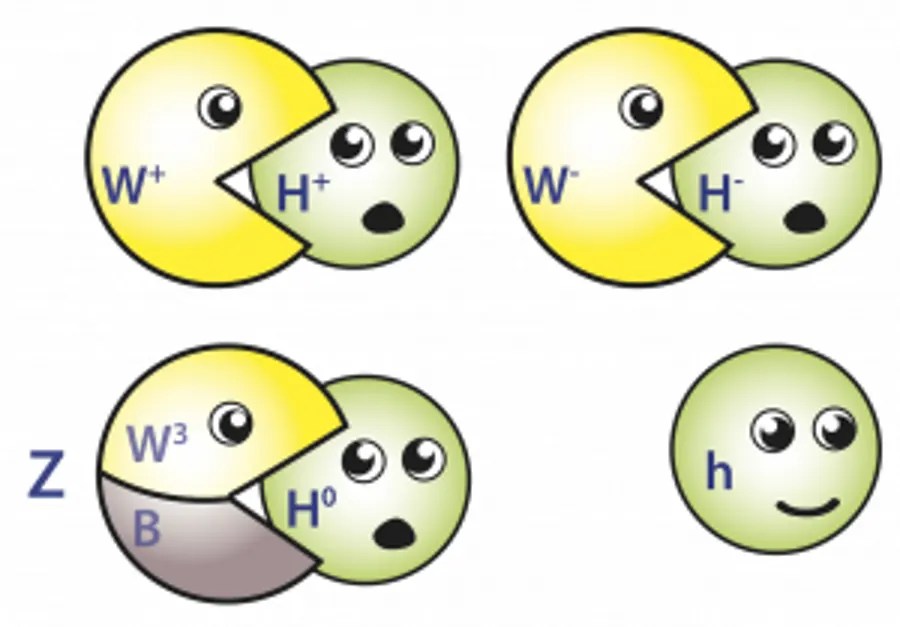
The Universe continues to expand and cool as it ages. By the time the Universe is just ten nanoseconds (10-8 seconds) old, it’s much cooler: just 100 trillion (1014) K, and it’s barely hot enough to continue creating the heaviest quarks and leptons: charm and bottom quarks and tau leptons, plus their antiparticle counterparts. The W-and-Z bosons and the Higgs bosons are all gone by this point, and we’re now firmly in territory that modern-day colliders have probed. However, temperatures and densities are still tremendous, and the Universe is in the phase of a quark-gluon plasma: where the energy and density of matter (and antimatter) particles are greater than what’s found inside an atomic nucleus.
As things continue to expand and cool on cosmic scales, the density of particles continues to drop and the temperature of particles drops as well. This makes it easier for still-heavier particles and antiparticles to decay than it is to create them. At around 20 nanoseconds, the b-quarks and antiquarks disappear, and then tau leptons and antileptons decay and disappear once around 100 nanoseconds have elapsed. Once 200 nanoseconds have passed, charm quarks and antiquarks go away as well, leaving only the three lightest quark/antiquark species along with two species of charged leptons and antileptons amidst a bath of photons, gluons, and neutrinos/antineutrinos. As things continue to cool, another critical “transition” happens once about 20 microseconds have elapsed: when the Universe is around a temperature of 3 trillion K.
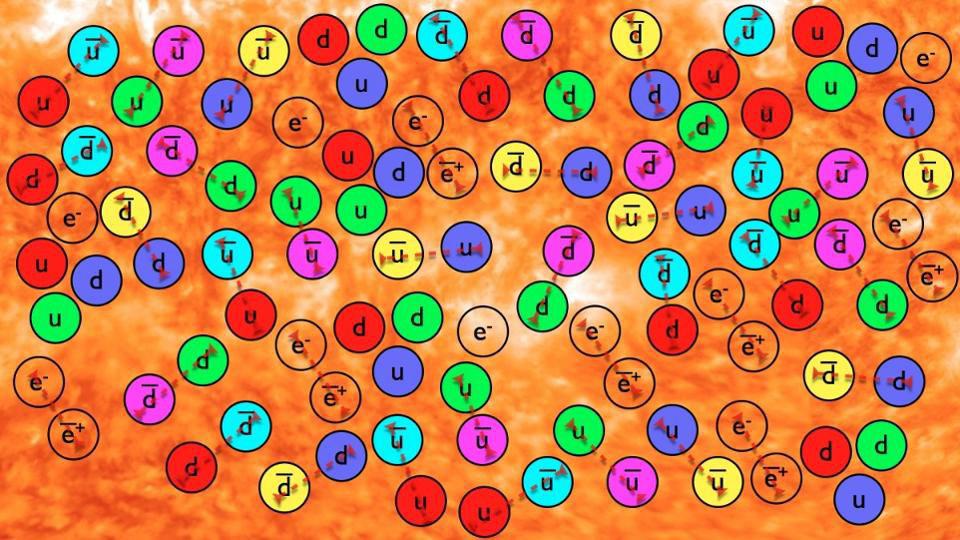
Known as the QCD phase transition, this is the crucial moment where the density and temperature of the Universe has dropped to sufficiently low values that instead of a sea of quarks and antiquarks and gluons, these subatomic particles condense to form hadrons: baryons (3 quarks), mesons (quark-antiquark combinations), and antibaryons (3 antiquarks) most prominently among them. Free quarks and antiquarks can no longer survive under these conditions; they must bind themselves together into these composite entities. Initially, there are tremendous numbers of baryons and antibaryons along with the mesons: nearly equal to photons and neutrinos (and antineutrinos) in their total, cumulative numbers.
Within another few microseconds, however, almost everything that has formed up until this point has now annihilated or decayed away. Particles that contain a strange quark or antiquark disappear first: in nanoseconds. The mesons also live for only nanoseconds, with the lightest (pions) turning primarily into muons and antimuons. Muons and antimuons annihilate away into photons, electron/positron pairs, and neutrino/antineutrino pairs, while the remainder decay with a mean lifetime of just 2.2 microseconds. And while there are initially enormous populations of baryons and antibaryons after this transition, they almost all annihilate away with their antiparticle counterparts.
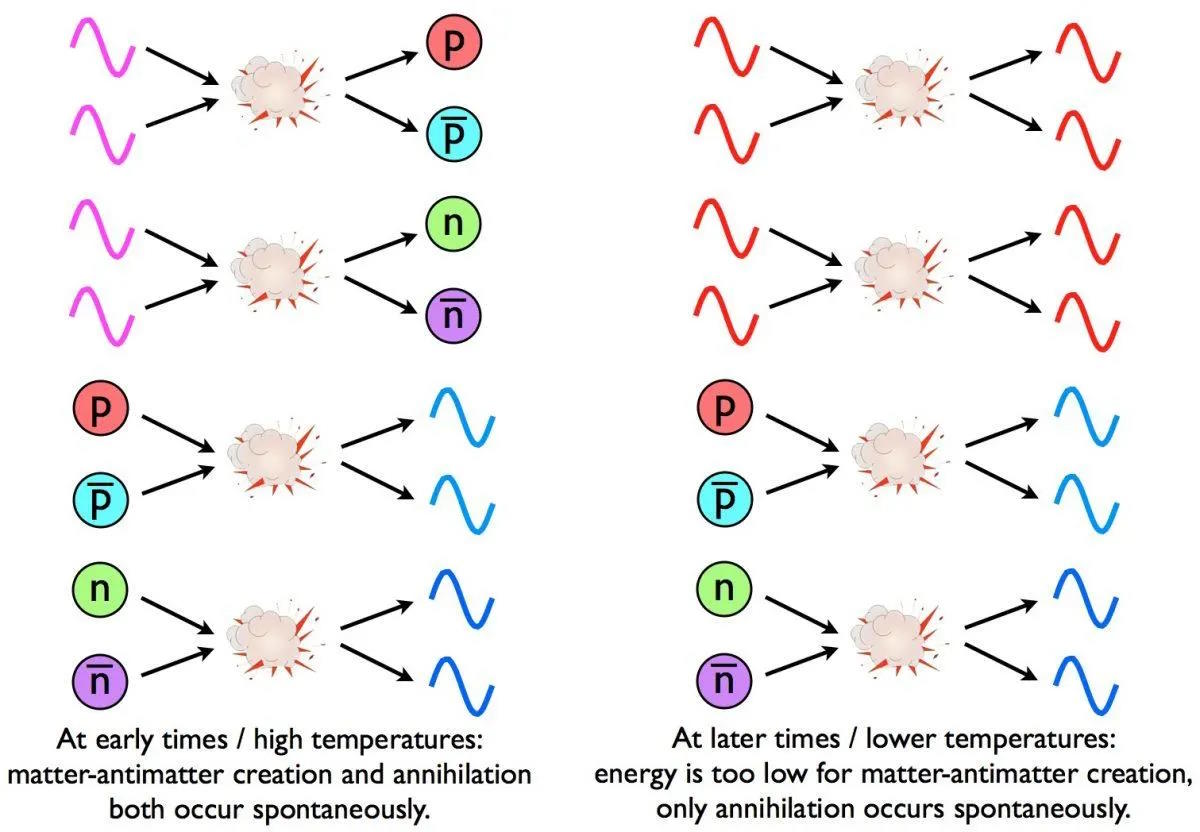
By the time the Universe is maybe ~30 microseconds old, there are only a small population of protons and neutrons left (in about a 50-50 ratio) amidst a sea of photons, neutrinos, antineutrinos, and electron-positron pairs. There are more than a billion photons for every single proton or neutron left, but there are no antiprotons or antineutrons remaining; a matter-dominated Universe has emerged from a state that was practically perfectly symmetrical between matter and antimatter. While a tiny excess of electrons over positrons also persists (keeping the Universe electrically neutral, with one “extra” electron for every proton that exists), it’s just as easy to create electron-positron pairs as it is for them to annihilate into photons and/or neutrino-antineutrino pairs.
Four important reactions occur in great numbers during this stage:
- protons collide with electrons, producing neutrons and a neutrino,
- protons collide with an antineutrino, producing neutrons and positrons,
- neutrons collide with neutrinos, producing protons and electrons,
- and neutrons collide with positrons, producing protons and an antineutrino.
For hundreds of milliseconds, these four reactions all occur in great abundance. But as the Universe cools further, down to a temperature of just 10 billion K or so, some of these reactions proceed more slowly than others.
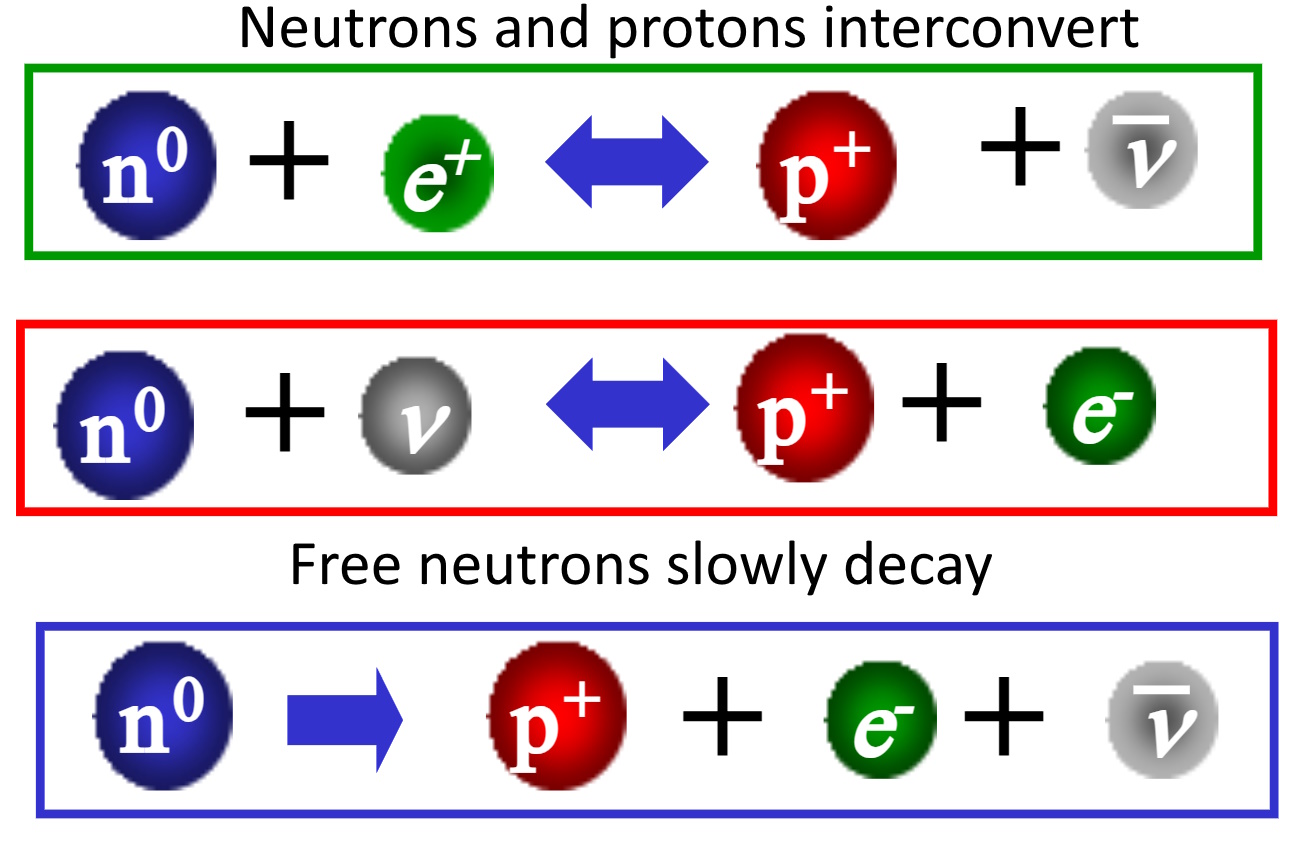
In particular, it becomes easier to collide neutrons with either neutrinos or positrons to create protons and either electrons or antineutrinos than the other way around. The reason for this is that the Universe has now cooled sufficiently so that most of the electrons or antineutrinos that exist, when a proton collides with them, doesn’t have enough energy to produce a neutron: a neutron is about 0.14% heavier than a proton, with that energy difference being more than double the rest-mass energy of an electron or positron. As a result, by the time the Universe reaches an age of about ~1 second, instead of protons and neutrons having a 50/50 split between them, the split has tilted to be more like 85/15 in favor of protons.
Now, the Universe continues to cool, and the weak interactions freeze out. Those protons colliding with electrons or antineutrinos no longer produce neutrons, and neutrinos and positrons that collide with neutrons no longer produce protons; the period of protons and neutrons interconverting into one another has ended. Around two seconds later, at an age of ~3 seconds, electrons and positrons annihilate faster than they can be created by the process of pair production via E = mc². Because the weak interactions have frozen out, they only annihilate to produce photons; they do not produce neutrinos or antineutrinos.
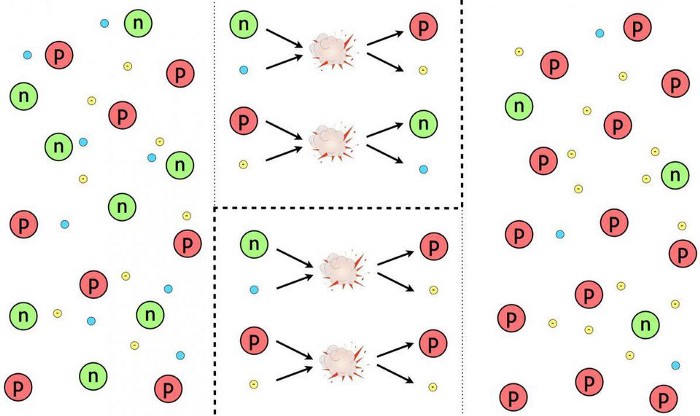
Now, at an age of ~3 seconds, our Universe contains:
- an enormous bath of photons, whose temperature is about 40% greater than
- the bath of neutrinos and antineutrinos, which also persist in enormous (but not quite-as-great) numbers,
- alongside a tiny number (less than 1 for every 1 billion photons) of baryons,
- where 85% of the baryons are protons and 15% of the baryons are neutrons,
- and where there’s one electron for every proton.
You might think about these tremendously dense conditions and the extreme energies (temperatures are still measured in the billions of degrees) and the fact that there are protons and neutrons just hanging out here, and expect that nuclear fusion will rapidly ensue, just as it does in the centers of modern-day stars.
It wants to! But there’s a problem: there are so many photons, about 1.6 billion of them for every baryon, that as soon as a proton and neutron react to form a deuteron, a sufficiently energetic photon comes along and blasts that nucleus apart. Deuterium is the lightest particle that is the product of nuclear fusion, and the first step in any chain-of-events that builds up heavier elements (or isotopes) in the periodic table. So long as there are enough photons of sufficient energy, deuterium is unstable, and fusion cannot proceed. (Cosmologists call this period of the early Universe “the deuterium bottleneck.”)

After about 20 seconds have elapsed, tiny, minuscule amounts of deuterium begin to survive for long enough to have other interactions with protons and neutrons, but the deuterium bottleneck persists for several minutes. During this time, the free, unbound protons remain stable, but the free, unbound neutrons are unstable: with a mean lifetime of just under 15 minutes. It isn’t until the Universe is nearly four minutes old that protons and neutrons fuse together in great number, but the decay of neutrons has further tilted the proton-to-neutron ratio: from 85/15 to more like 88/12.
Finally, nuclear fusion proceeds in earnest: producing lots of helium-4, smaller amounts of deuterium and helium-3, and minuscule amounts of lithium and still-heavier elements. Our earliest atomic nuclei have formed. It will take hundreds of thousands of years for those atomic nuclei to stably combine with electrons to make neutral atoms, as the temperature of the Universe must cool from the billions of degrees down to just a few thousand degrees: the sufficiently low temperatures that allow atoms to exist in a neutral state, rather than in a state of ionization. It’s only after this final transition, some 380,000 years after the Big Bang began, that neutral atoms can form and gravitationally collapse, eventually leading to stars and our modern cosmos.

But at each step along the way, the Universe expanded and cooled, undergoing many “step downs” in energy, including through some remarkable transitions, to create the Universe we recognize. Heavy, unstable particles were created early on, but as the energy dropped, their creation ceased, forcing them to either annihilate or decay away. Symmetries broke, enabling the particles of the Standard Model to acquire a rest mass and separating the forces into four fundamental ones. Quarks and antiquarks and gluons formed hadrons like mesons and baryons and antibaryons, and even lighter unstable particles decayed away.
Finally, protons and neutrons interconverted, that interconversion ceased as the weak interactions became cosmically unimportant, and then electrons and positrons annihilated away, increasing the photon temperature relative to the neutrino/antineutrino temperature. Nuclear fusion occurred in a brief burst, giving rise to the light elements, and very slowly and gradually, the Universe cooled sufficiently so that neutral atoms could form. Then, on cosmic timescales, gravitational collapse occurred, giving rise to stars, galaxies, heavy elements, rocky planets, a cosmic web, and eventually life. It’s taken us this long to reconstruct this much of our cosmic history, with many details of these later stages and nearly all details of the very earliest stages still awaiting our discovery.
However, with each “transition” down the energy staircase, an important aspect of our modern Universe came into focus. From a uniform sea of primordial chaos, our ordered Universe, dominated by atoms, has emerged.