Why the electron’s mass is vital to life in the Universe
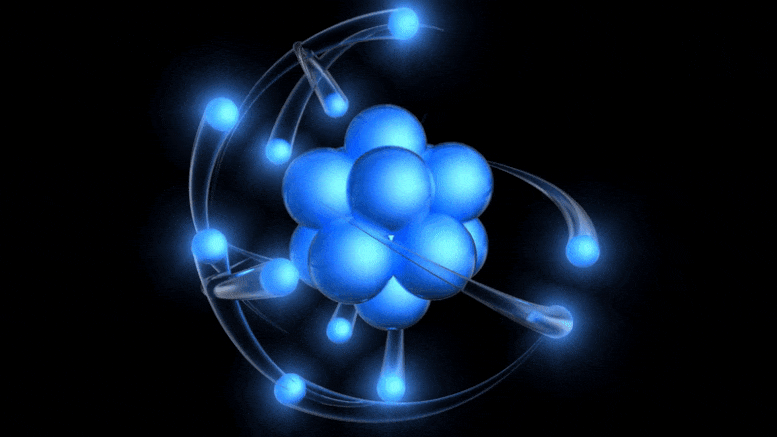
- The properties of our Universe intimately depend on the values of fundamental constants: the speed of light, the strength of the gravitational force, Planck’s constant, the masses of the fundamental particles, and more.
- For most of these properties, we could increase or decrease the values of those constants significantly, and the Universe would remain mostly the same: with stars, galaxies, and the potential for life.
- However, one fundamental constant that we couldn’t change by very much is the mass of the electron, otherwise complex molecules, and life, would never be possible. Here’s why.
One of the biggest puzzles we face about the Universe is that we have no explanation for a great many of the properties that the fundamental objects within it possess. There are four fundamental forces, each with their own strength of interaction, and there’s no explanation for why those forces have both the absolute and relative strengths that they possess. Some particles possess an electric charge, and we have no explanation for why the charge of the electron, proton, or any of the quarks have the values that they do. And although the Higgs provides the fundamental particles with the rest masses that they possess, we have no explanation for why those masses have the values that they do. Other constants of nature, such as the speed of light, also come along without explanation.
All told, it takes at least 26 separate fundamental constants to describe the Universe that we presently understand, and we have no idea why these constants have the values that they do. If some of these constants were either too small or too large, our Universe as we know it would be impossible; our very existence is evidence that the laws of nature must be consistent with our existence being possible. If gravity were a little bit stronger or weaker, stars, galaxies, planets, and life would still exist. Same with:
- the strengths of the other forces,
- the masses of the quarks,
- or the value of the speed of light.
Sure, atoms might have slightly different sizes, light signals might take different amounts of time to propagate, and the types of large-scale structures that exist might be somewhat different in detail, but it would still be a Universe where life like us was possible.
But that wouldn’t be the case if the electron’s mass was different. Here’s the surprising science of why.
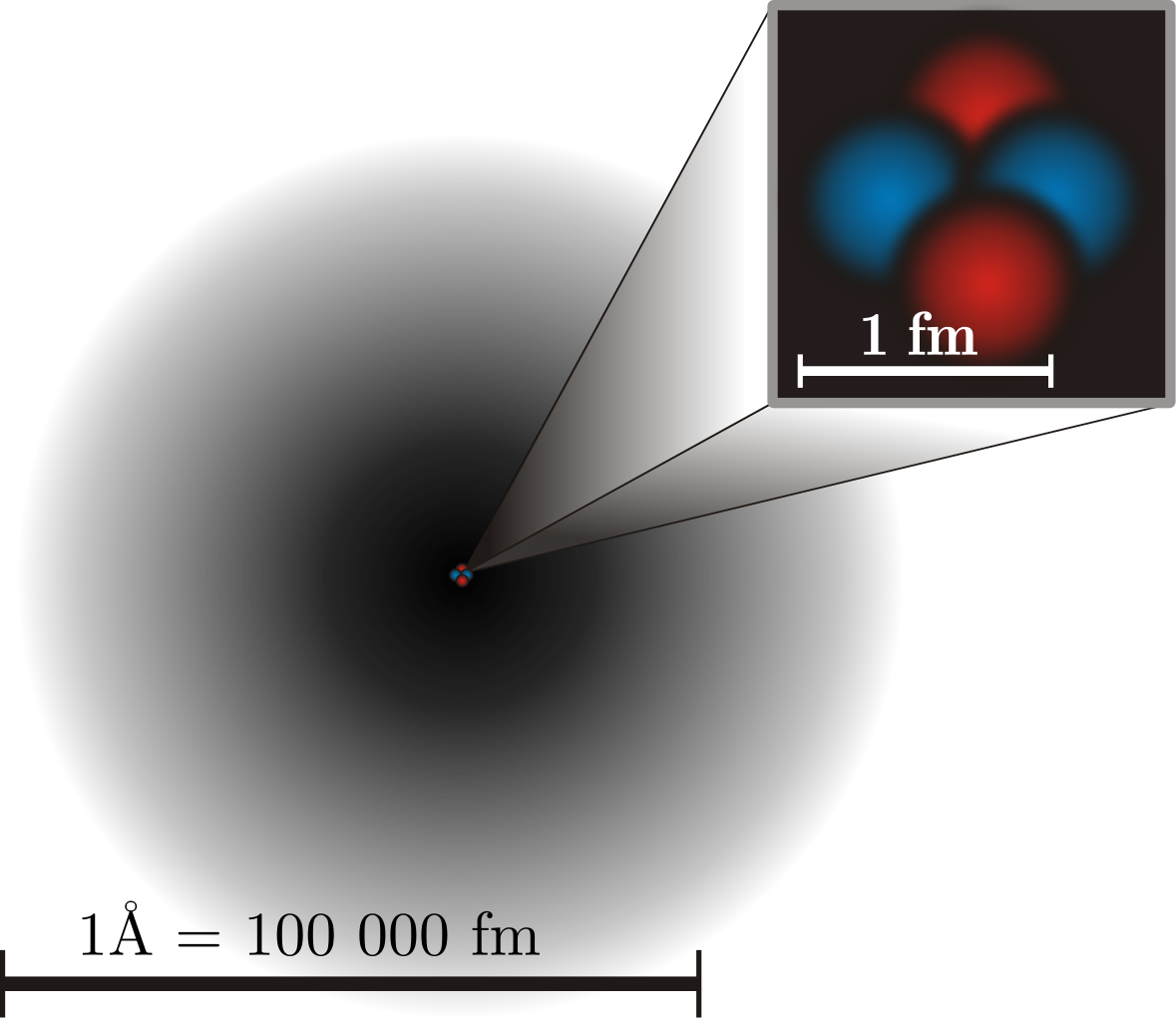
When it comes to our own selves, there’s no denying what we’re made up of: atoms. At the center of every atom is a small, compact, massive, and positively charged atomic nucleus: something containing over 99.9% of an atom’s mass, but that’s incredibly tiny, with a size that’s measured in femtometers, where 1 femtometer is a minuscule 10-15 meters. Orbiting those atomic nuclei are electrons: even smaller particles that are, by contrast, very light and low in mass, but that have a negative charge to them: a charge that’s equal-and-opposite to the charge on every proton. We’ve smashed electrons into other electrons and a variety of other particles inside giant particle accelerators, and have determined that if there is a physical “size” to them, they’re at least smaller than 10-19 meters. As far as we can tell, they may be truly point-like.
However, the size of an atom itself is much larger than the size of its components. Protons and neutrons bind together to make atomic nuclei, and then each atom — typically — contains an equal number of electrons to the number of protons in its nucleus, making something that’s electrically neutral. Whereas electrons are point-like (or, at least, no larger than 10-19 meters) and atomic nuclei are on the scale of femtometers (10-15 meters), atoms themselves are normally measured in units of angstroms, where one angstrom is an impressively large 10-10 meters, or some 100,000 times larger than the atom’s nucleus and more than a billion times larger than the physical size of the electron itself.

Our existence depends on these atoms. Inside the human body, if you were to count up every proton, neutron, and electron making ourselves up, you’d wind up with an enormous number: a number that exceeded 1029, also known as 100 octillion. These are bound up together in the form of atoms, with the most common atom being hydrogen, which was predominantly formed in the Big Bang. However, there are around 90 different species of atoms that naturally occur in this Universe — known as elements — with the specific “species” of atom determined by the total number of protons within its atomic nucleus.
Several dozens of these species of atoms are required to compose a full-fledged human being, with oxygen and carbon atoms making up the majority of a human’s mass. Also vital to a human’s existence are the elements:
- nitrogen,
- calcium,
- phosphorus,
- potassium,
- sulfur,
- sodium,
- chlorine,
- magnesium,
- and iron,
which, all added together with oxygen, carbon, and hydrogen, compose 99.9% of an average human’s mass. With the exception of hydrogen, which was formed in the Big Bang, all of these other, heavier elements were formed in stars or from the aftermath of stellar processes. As long as we don’t tinker with the fundamental constants too much, we’ll still have stars, nuclear fusion, and the creation of all of these heavy elements.

This is part of the standard story of how life arose in our Universe. Our Universe as we know it began with the hot Big Bang, and in the aftermath of that event, everything expanded, cooled, became less dense, and gradually became non-uniform as it clumped and clustered together under the influence of gravity. Early on, protons and neutrons formed from quarks and gluons: something that would have been possible even if quarks remained entirely massless. If you increased or decreased the mass of the quarks by a factor of 10 or even 100, the masses of the proton and neutron would change very little. That’s because the mass of the quarks is tiny relative to the mass of the proton: making up only around ~1% of the proton’s total mass. Even if the quarks were all completely massless, the majority of the proton’s mass comes from the gluon field — i.e., the strength of the strong force — and so tinkering with quark masses only changes the proton’s mass very slightly.
A little later, nuclear fusion occurred, creating the light atomic nuclei like helium and lithium. Nuclear fusion would rear its head again millions of years later, once stars began forming from dense collections of gravitationally collapsed matter. Again, even substantial changes to the fundamental constants wouldn’t forbid this from occurring, enabling the Universe to be littered with the heavy elements formed in stars: carbon, oxygen, nitrogen, and a whole lot more. Once the interstellar medium becomes sufficiently enriched, the next generation of stars to form will be rich enough in heavy elements that rocky planets (or rocky moons around gas giant planets) can form, raising the possibility that life can finally arise in the Universe.
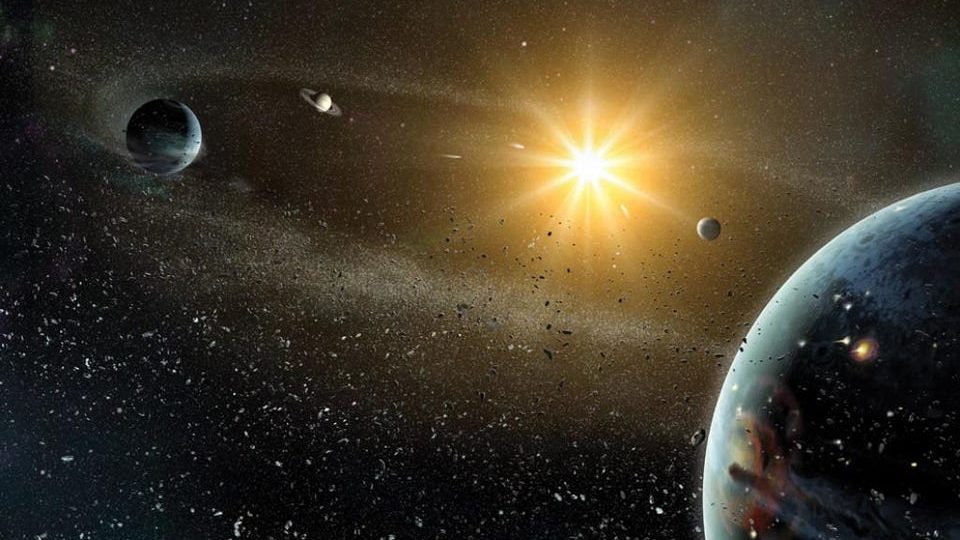
Almost any fundamental constant — the speed of light, the gravitational constant, Planck’s constant, the masses of the quarks, etc. — could be changed dramatically, turned way up or way down, and the broad strokes of this cosmic story would still remain intact. But if you tried to tinker with the mass of the electron in this fashion, the possibility of life arising swiftly vanishes.
- If you were to raise the mass of the electron by too great of an amount, atomic and molecular transitions would become impossible under conventional conditions, even in direct sunlight.
- Similarly, if you were to lower the mass of the electron significantly, even weak, low-energy interactions would prevent us from having stable atoms or molecules of any type for very long.
It’s only with the value of the mass of the electron our Universe actually has, or at least with that mass falling in a very narrow range, that life, organic molecules, or even complex chemistry of any type remains possible. We can understand this by comparing the most common form of hydrogen that we know of, where a single proton is orbited by a single electron, with a special “exotic” species of hydrogen that can only be made very briefly under laboratory conditions: muonic hydrogen.
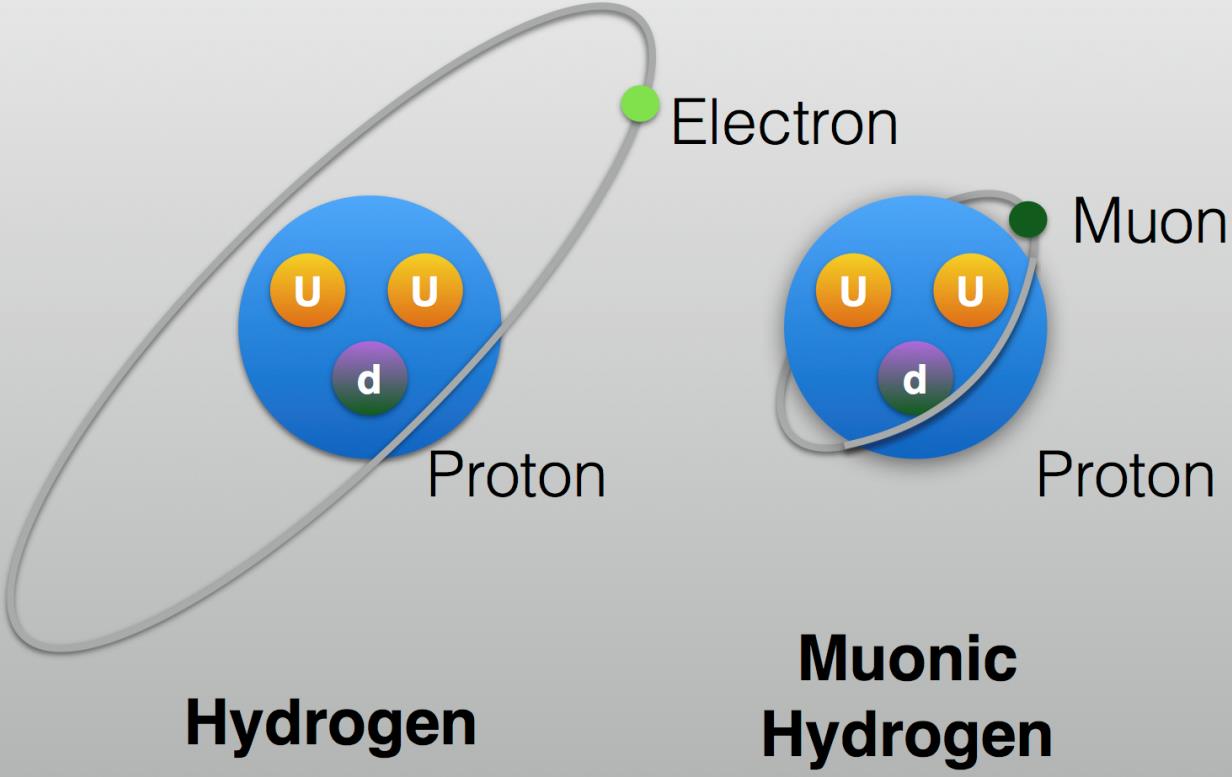
While conventional, normal matter is made of protons, neutrons, and electrons, those aren’t the only possibilities that exist when it comes to matter. Protons and neutrons are made out of up-and-down quarks, but four other, heavier species of quark exist: the strange, charm, bottom, and top quarks. Electrons may be the lightest charged particle, but it has two heavier, unstable cousins: the muon and the tau. Whereas individual protons and electrons are stable (as well as nuclei made out of both protons and neutrons combined), none of the other types of matter that exist — matter made up of heavier quarks or matter containing muons or taus — will remain in existence for very long. Because of the weak nuclear interaction, they’ll swiftly decay away into lighter, more stable “daughter” particles.
However, the muon is the longest-lived of the unstable fundamental particles, persisting with a mean lifetime for a whopping 2.2 microseconds. This might not seem like a long time at all, but it’s long enough for muons to form bound states with protons, creating an exotic form of hydrogen that’s different in a number of important ways. The muon, like the electron, is:
- a lepton,
- with a charge that’s equal and opposite to the proton’s,
- and that’s significantly lighter than the proton itself.
However, compared to the electron, the muon has a mass that’s more than 200 (precisely, 206) times as great, while maintaining the same electric charge. That heavier mass means that the electric force, which keeps electrons from flying away from the atomic nucleus, confines the muon to be much, much closer to the atomic nucleus than an electron can be.
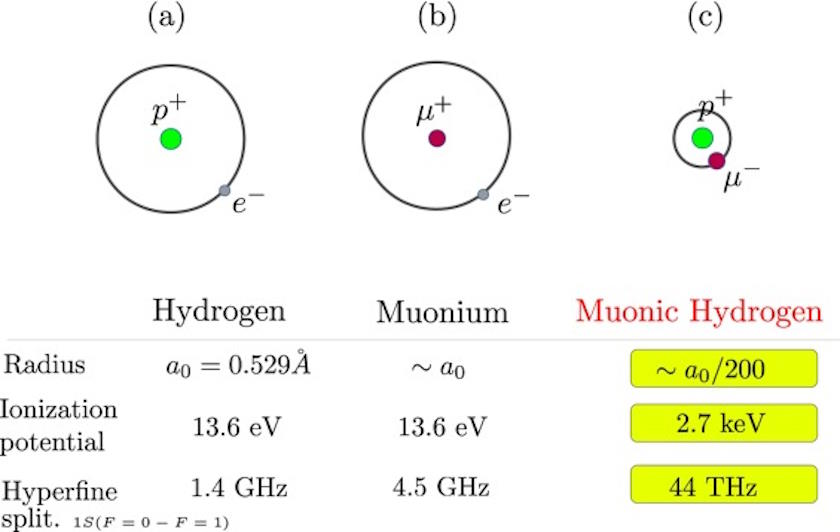
The ratio turns out to be direct: for a muon, a particle that’s 206 times as massive as the electron but with the same electric charge, the radius of muonic hydrogen is 1/206th the radius of standard hydrogen. Instead of a normal hydrogen atom, which is about 1 angstrom (10-10 meters) in size, an atom of muonic hydrogen is only about 0.005 angstroms (5 × 10-13 meters) in size. Muons, being heavier than electrons, are unstable, and will spontaneously decay into an electron, an electron antineutrino, and a muon neutrino after a few microseconds. However, for as long as that muon persists and muonic hydrogen remains stable, there are a number of important differences between it and standard hydrogen.
Perhaps the biggest difference is in terms of energy levels. Normal (electron-containing) hydrogen has only a specific set of energy levels that it can occupy: the ground state (n=1), the first excited state (n=2), all the way up to a fully ionized state (n=∞), and in order to rise up in energy level, a photon of the proper energy needs to interact with it. To go from the ground (lowest-energy) state up to the first excited state, an energy of 10.2 eV (electron-volts) is needed; to fully ionize, an energy of 13.6 eV is needed. Because a muon has 206 times the mass of an electron, it takes 206 times the energy. Muonic hydrogen can’t become excited unless a photon of 2.1 keV (or kilo-electron-volts) strikes it, and can’t become ionized unless a photon of at least 2.8 keV encounters it.
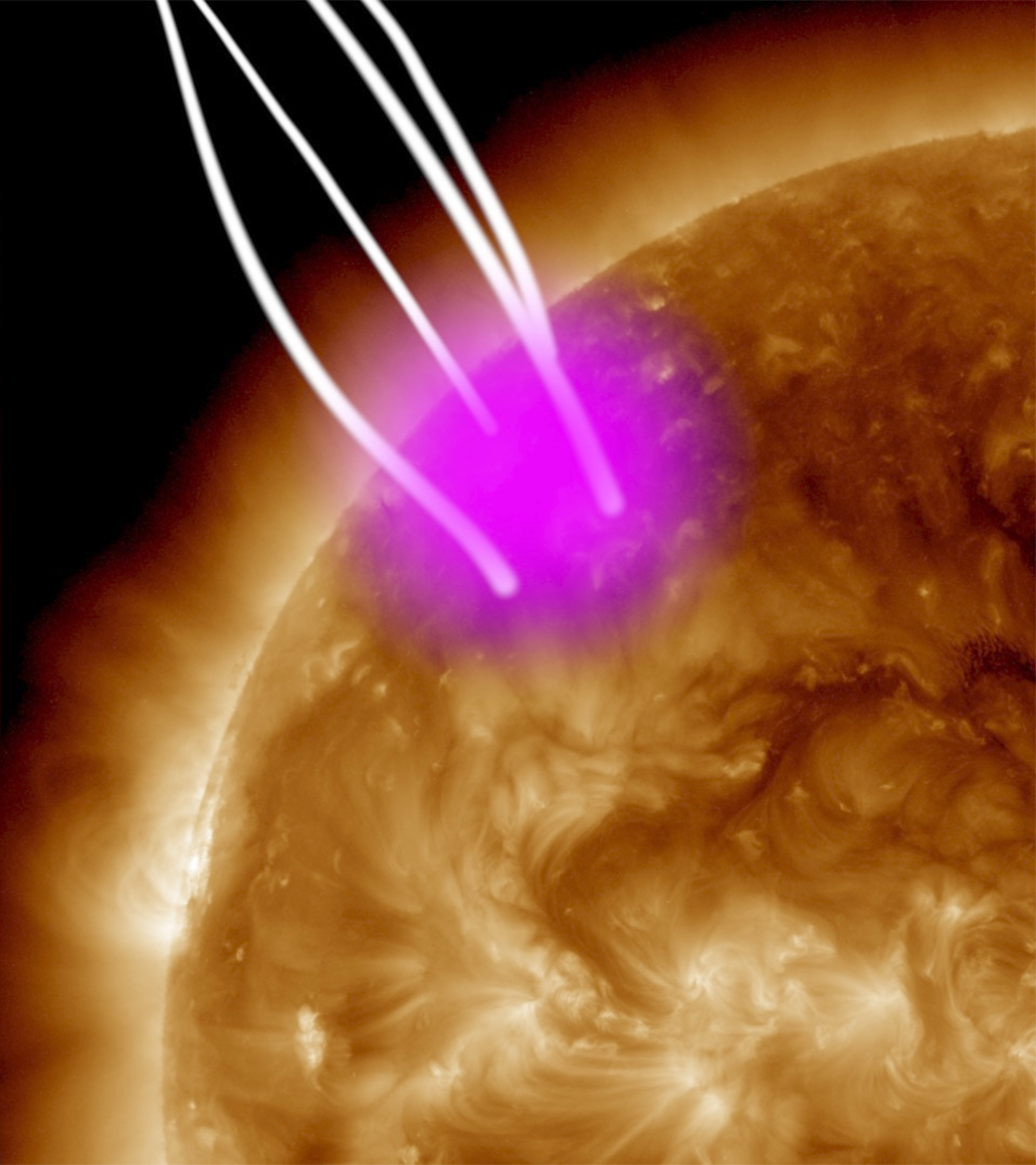
We have photons of this energy in our Universe, but they’re rare: they’re examples of X-rays. Stars like the Sun emit X-rays, but only in very tiny amounts: about one-trillionth of the amount of energy as is emitted in visible light. Whereas sunlight powers all sorts of atomic and molecular processes on Earth, including the incredibly biologically important photosynthesis, it’s because of the enormous amount of solar energy that strikes the Earth that life is possible. The Sun imparts a total of around 1500 watts of power to every square meter of Earth that it strikes, with most of that energy coming in the form of visible, near-infrared, and ultraviolet light. Only about ten nanowatts of that power (10-8 W) exists in the form of X-rays.
In other words, if the electron has a significantly higher rest mass than it does, the chemistry-based reactions that power all biological processes on Earth would be extraordinarily rare, as the energetic events that occur in the Universe — the shining of stars, geothermal heat, volcanic eruptions, etc. — would only rarely even be able to cause an atomic or molecular transition. Without those, no sorts of complex chemistry, chain reactions, or biological processes would be able to reliably occur, much less occur readily and ubiquitously. A Universe where the electron was heavier than it is, even by a factor of 10 or so (and perhaps even less), would be incapable of supporting life as we know and understand it.

The opposite problem would arise if the electron were too light. Just as a heavier electron would mean a smaller, more tightly bound, and more challenging-to-excite (or ionize) atom, a lighter electron would translate into the opposite set of conditions: a larger, more loosely bound, more easily excited (or ionized) atom. As in the case of a heavier electron, this wouldn’t apply only to hydrogen, but to any and all atoms.
Now consider that the average energy of a visible light photon — the kind produced by the Sun and all stars undergoing nuclear fusion in their cores — is around 2 or 3 eV. If we reduced the mass of the electron even by a factor of five, to just 20% of its present mass, then instead of atomic or molecular transitions occurring frequently in direct sunlight, atomic and molecular bonds would be routinely destroyed, as those atoms and molecules would be ionized completely, simply by exposing them to light.
Sunlight has the power to create but also to destroy. It’s only because the mass of the electron falls into that “sweet spot” where atomic and molecular transitions are routinely stimulated by, but where those bonds are not broken by, the energy of direct sunlight that so many reactions, including photosynthetic ones, are possible and routine: on our world and within our Universe.
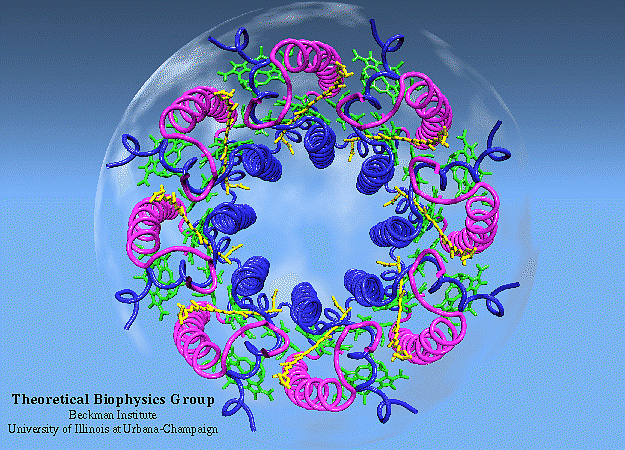
One of the questions people often ask, when looking at the laws of nature and the fundamental constants that describe it, is whether our Universe is fine-tuned for our existence to be admissible. When it comes to most of the laws and most of the constants, it’s generally understood that increasing or decreasing the strengths of interactions or the values of the constants would tweak certain specifics about our Universe, but that it would still very much resemble the Universe we know. Even if we changed things by a great deal — a factor of 10, 100, 1000, or even more — while our Universe would have noticeable differences, things that we’re familiar with, like atoms, molecules, stars, black holes, nuclear reactions, planets, galaxies, and even chemistry and life, would still likely exist in some form.
But the building block structures of the small-scale objects in our Universe, i.e., atoms, are extremely sensitive to the mass of the electron. If everything else remained the same but the mass of the electron were at all substantially different from the value it possesses today — whether significantly lighter or heavier — complex chemistry and life processes would be all but forbidden. Too light of an electron would lead to a Universe where atoms and molecules were too easily destroyed, and where even visible light would “cook” anything that attempted to form. Too heavy of an electron, and atoms and molecules couldn’t leave the ground state, unable to undergo the types of transitions that all chemical and biological reactions depend upon.
Life is certainly possible within our Universe and with the constants that we have. But if the electron’s mass were altered only slightly — either heavier or lighter — the Universe would be a whole lot lonelier.