Ask Ethan: How does the CMB prove the Big Bang?
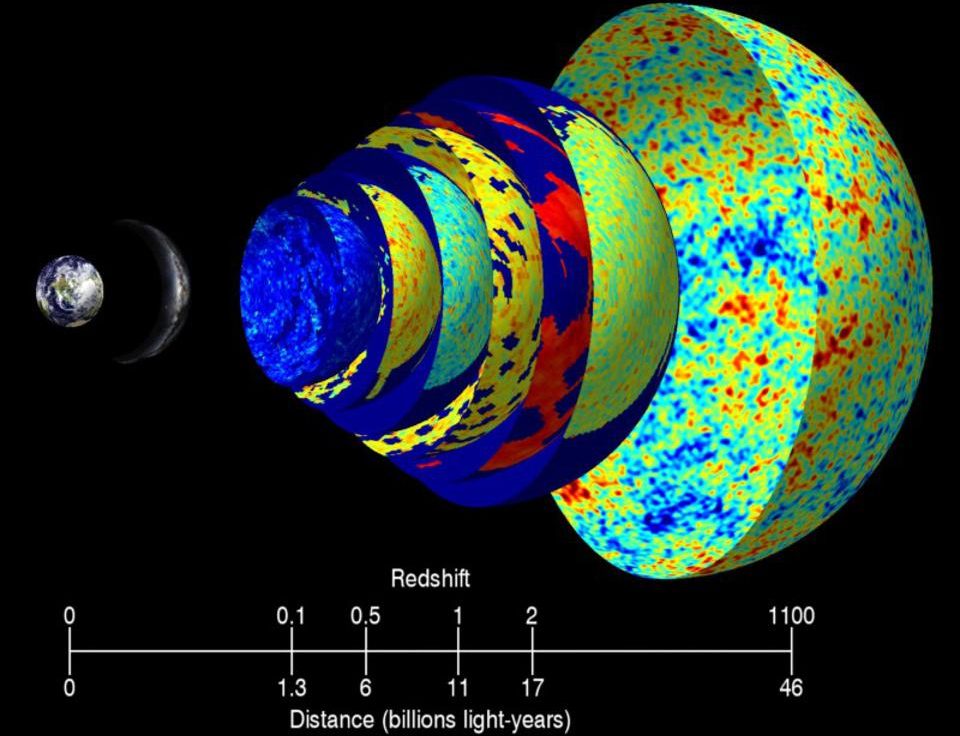
- Since time immemorial, humans have wondered what the Universe is, where it came from, and how it got to be the way it is today.
- Once a question far beyond the realm of knowledge, science was finally able to settle many of these puzzles in the 20th century, with the cosmic microwave background providing the critical evidence.
- There’s a set of compelling reasons why the hot Big Bang is now our undisputed cosmic origin story, and this leftover radiation is what decided the issue. Here’s how.
Less than a century ago, we had many different ideas for what the history of our Universe looked like, but shockingly little evidence available to decide the issue. Hypotheses included suggestions that our Universe:
- violated the principle of relativity, and that the light we observed from distant objects simply got tired as it traveled through the Universe,
- was the same not only in all locations, but at all times: static and unchanging even as our cosmic history unfolded,
- didn’t obey general relativity, but rather a modified version of it that included a scalar field,
- didn’t include ultra-distant objects, and that those were nearby interlopers that observational astronomers were confounding for distant ones,
- or that it began from a hot, dense state and had been expanding and cooling ever since.
That last example corresponds to what we know today as the hot Big Bang, while all the other challengers (including newer ones not mentioned here) have fallen by the wayside. Since the mid-1960s, in fact, no other explanation has held up to the observations. Why is that? That’s the inquiry of Roger Brewis, who would like some information about the following:
“You cite the blackbody spectrum of the CMB as confirmation of the Big Bang. Could you tell me where I can get more detail on this, please.”
There’s never anything wrong with asking for more information. It’s true: the cosmic microwave background (CMB) radiation, which we’ve concluded is the leftover glow from the Big Bang itself, is that key evidence. Here’s why it confirms the Big Bang, and disfavors all other possible interpretations.

There were two developments in the 1920s that, when combined, led to the original idea that would eventually evolve into the modern Big Bang theory.
- The first was purely theoretical. In 1922, Alexander Friedmann found an exact solution to Einstein’s equations in the context of general relativity. If one constructs a Universe that’s isotropic (the same in all directions) and homogeneous (the same in all locations), and fills that Universe with any combination of various forms of energy, the solution showed that the Universe could not be static, but must always either expand or contract. Furthermore, there was a definitive relationship between how the Universe expanded over time and the density of energy within it. The two equations derived from his exact solutions, the Friedmann equations, are still known as the most important equations in the Universe.
- The second was based on observations. By identifying individual stars and measuring the distance to them in spiral and elliptical nebulae, Edwin Hubble and his assistant, Milton Humason, were able to show that these nebulae were actually galaxies — or, as they were then known, “island universes” — beyond our Milky Way. Additionally, these objects appeared to be receding from us: the farther away they were, the faster they appeared to recede.

Combine these two facts, and it’s easy to come up with the idea that would lead to the Big Bang. The Universe could not be static but must be either expanding or contracting if general relativity is correct. Distant objects appear to be receding from us, and receding faster the farther they are from us, suggesting the “expanding” solution is physically relevant. If this is the case, then all we have to do is measure what the various forms and densities of energy in the Universe are — along with how quickly the Universe is expanding today and was expanding at various epochs in the past — and we can practically know it all.
We can know what the Universe is made of, how fast it’s expanding, and how that expansion rate has (and therefore, the various forms of energy density have) changed over time. Even if you assumed that all that’s in the Universe is what you can easily see — things like matter and radiation — you’d reach a very simple, straightforward conclusion. The Universe, as it is today, isn’t just expanding but is also cooling, as the radiation within it is getting stretched to longer wavelengths (and lower energies) by the expansion of space. That means, in the past, the Universe must have been smaller, hotter, and denser than it is today.
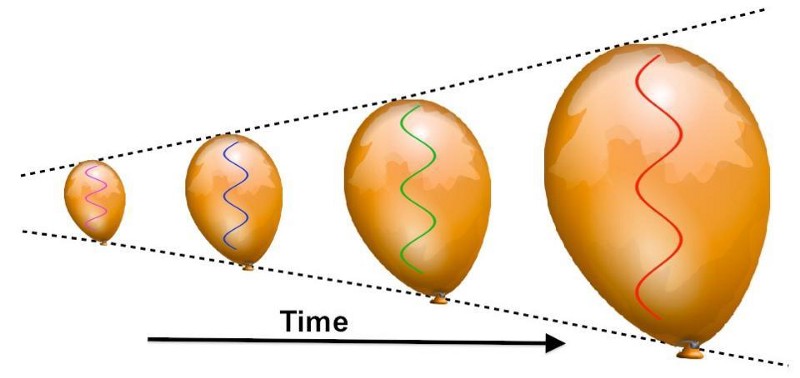
Extrapolating backward, you’d begin to make predictions for how the Universe should have appeared in the distant past.
- Because gravitation is a cumulative process — larger masses exert a greater amount of gravitational attraction across larger distances than smaller masses do — it makes sense that the structures in the Universe today, like galaxies and galaxy clusters, grew up from smaller, lower-magnitude seeds. Over time, they attracted more and more matter into them, leading to more massive and more evolved galaxies appearing at later times.
- Because the Universe was hotter in the past, you can imagine a time, early on, when the radiation within it was so energetic that neutral atoms couldn’t have stably formed. The instant an electron tried to bind to an atomic nucleus, an energetic photon would come along and ionize that atom, creating a plasma state. Therefore, as the Universe expanded and cooled, neutral atoms stably formed for the first time, “releasing” a bath of photons (that would have previously scattered off of free electrons) in the process.
- And at even earlier times and hotter temperatures, you can imagine that not even atomic nuclei could have formed, as the hot radiation would have simply created a sea of protons and neutrons, blasting any heavier nuclei apart. Only when the Universe cooled through that threshold could heavier nuclei have formed, leading to a set of physical conditions that would have formed a primitive set of heavy elements through nuclear fusion occurring in the aftermath of the Big Bang itself.

These three predictions, along with the already-measured expansion of the Universe, now form the four modern cornerstones of the Big Bang. Although the original synthesis of Friedmann’s theoretical work with the observations of galaxies occurred in the 1920s — with Georges Lemaître, Howard Robertson, and Edwin Hubble all putting together the pieces independently — it wouldn’t be until the 1940s that George Gamow, a former student of Friedmann, would put forth these three key predictions.
Early on, this idea that the Universe began from a hot, dense, uniform state was known as both the “cosmic egg” and the “primeval atom.” It wouldn’t pick up the name “Big Bang” until a proponent of the Steady State theory and derisive detractor of this competing theory, Fred Hoyle, gave it that moniker on BBC radio while passionately arguing against it.
Meanwhile, however, people began working out specific predictions for the second of these novel predictions: what this “bath” of photons would look like today. Back in the early stages of the Universe, photons would exist amidst a sea of ionized plasma particles: atomic nuclei and electrons. They would collide with these particles constantly, particularly the electrons, thermalizing in the process: where the massive particles achieve a particular energy distribution that’s simply the quantum analogue of a Maxwell-Boltzmann distribution, with the photons winding up with a particular energy spectrum known as a blackbody spectrum.

Prior to the formation of neutral atoms, these photons exchange energy with the ions throughout empty space, achieving that blackbody spectral energy distribution. Once neutral atoms form, however, these photons no longer interact with them, as they don’t have the right wavelength to be absorbed by the electrons within atoms. (Remember, free electrons can scatter with photons of any wavelength, but electrons within atoms can only absorb photons with very specific wavelengths!)
As a result, the photons simply travel throughout the Universe in a straight line, and will continue to do so until they run into something that absorbs them. This process is known as free-streaming, but the photons are subject to the same process that all objects traveling through the expanding Universe must contend with: the expansion of space itself.
As the photons free-stream, the Universe expands. This both dilutes the number density of photons, as the number of photons remains fixed but the volume of the Universe increases, and also decreases the individual energy of each photon, stretching each one’s wavelength by the same factor as the Universe expands.

That means, remaining today, we should see a leftover bath of radiation. With lots of photons for every atom in the early Universe, neutral atoms would only have formed once the temperature of the thermal bath cooled to a few thousand degrees and would have taken hundreds of thousands of years after the Big Bang to get there. Today, billions of years later, we’d expect:
- that leftover bath of radiation should still persist,
- it should be the same temperature in all directions and at all locations,
- there should be somewhere around hundreds of photons in every cubic centimeter of space,
- it should only be a few degrees above absolute zero, shifted into the microwave region of the electromagnetic spectrum,
- and, perhaps most importantly, it should still maintain that “perfect blackbody nature” to its spectrum.
In the mid-1960s, a group of theorists at Princeton, led by Bob Dicke and Jim Peebles, were working out the details of this theorized leftover bath of radiation: a bath that was then known poetically as the primeval fireball. Contemporaneously, and quite by accident, the team of Arno Penzias and Robert Wilson found the evidence for this radiation using a new radio telescope — the Holmdel Horn Antenna — located just 30 miles away from Princeton.

Originally, there were only a few frequencies that we could measure this radiation at; we knew it existed, but we couldn’t know what its spectrum was: how abundant photons of slightly different temperatures and energies were relative to one another. After all, there could be other mechanisms for creating a background of low-energy light throughout the Universe.
- One rival idea was that there were stars all throughout the Universe, and had been for all of time. This ancient starlight would be absorbed by interstellar and intergalactic matter, and would re-radiate at low energies and temperatures. Perhaps there was a thermal background from these radiating dust grains.
- Another rival, related idea is that this background simply arose as being reflected starlight, shifted toward lower energies and temperatures by the expansion of the Universe.
- Still another is that an unstable species of particle decayed away, leading to an energetic background of light that then cooled to lower energies as the Universe expanded.
However, each one of these explanations comes along with its own distinct prediction for what the spectrum of that low-energy light should look like. Unlike the true blackbody spectrum arising from the hot Big Bang picture, however, most of them would be the sum of light from a number of different sources: either throughout space or time, or even a number of different surfaces originating from the same object.
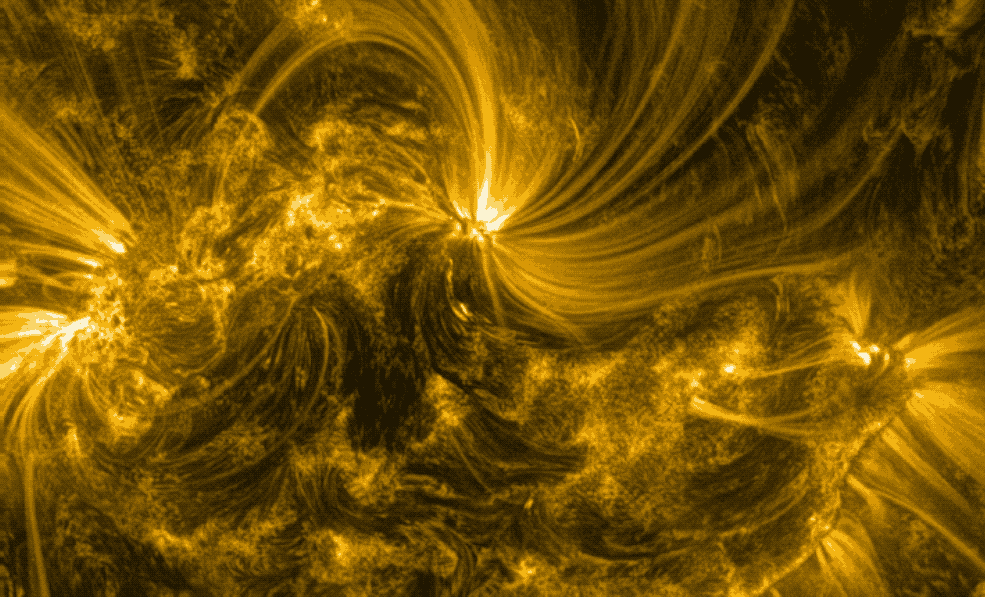
Consider a star, for example. We can approximate our Sun’s energy spectrum by a blackbody, and it does a pretty good (but imperfect) job. In truth, the Sun isn’t a solid object, but rather a large mass of gas and plasma, hotter and denser toward the interior, and cooler and more rarified toward the exterior. The light we see from the Sun isn’t emitted from one surface at the edge, but rather from a series of surfaces whose depths and temperatures vary. Instead of emitting light that’s one single blackbody, the Sun (and all stars) emit light from a series of blackbodies whose temperatures vary by hundreds of degrees.
Reflected starlight, as well as absorbed and re-emitted light, as well as light that’s created at a series of times instead of all-at-once, all suffer from this problem. Unless something comes along at some later time to thermalize these photons, putting all the ones from all across the Universe into the same equilibrium state, you won’t get a true blackbody.
And although we had evidence for a blackbody spectrum that improved greatly throughout the 1960s and 1970s, the biggest advance came in the early 1990s, when the COBE satellite — short for COsmic Background Explorer — measured the spectrum of the Big Bang’s leftover glow to greater precision than ever. Not only is the CMB a perfect blackbody, it’s the most perfect blackbody ever measured in the entire Universe.

Throughout the 1990s, 2000s, the 2010s, and now into the 2020s, we’ve measured the light from the CMB to greater and greater precision. We’ve now measured temperature fluctuations down to about 1-part-per-million, discovering the primordial imperfections imprinted from the inflationary stage that preceded the hot Big Bang. We’ve measured not just the temperature of the CMB’s light, but also its polarization properties. We’ve begun to correlate this light with the foreground cosmic structures that have formed subsequently, quantifying the latter’s effects. And, along with the CMB evidence, we now have confirmation of the other two cornerstones of the Big Bang as well: structure formation and the primordial abundance of the light elements.
It’s true that the CMB — which I honestly wish still had as cool a name as “the primeval fireball” — provides incredibly strong evidence in support of the hot Big Bang, and many alternative explanations for it fail spectacularly. There isn’t just a uniform bath of omnidirectional light coming toward us at 2.7255 K above absolute zero, it also has a blackbody spectrum: the most perfect blackbody in the Universe. Until an alternative can not only account for this evidence, but also the other three cornerstones of the Big Bang, we can safely conclude there are no serious competitors to our standard cosmological picture of reality.
Send in your Ask Ethan questions to startswithabang at gmail dot com!