Ask Ethan: Do we know why the Big Bang really happened?
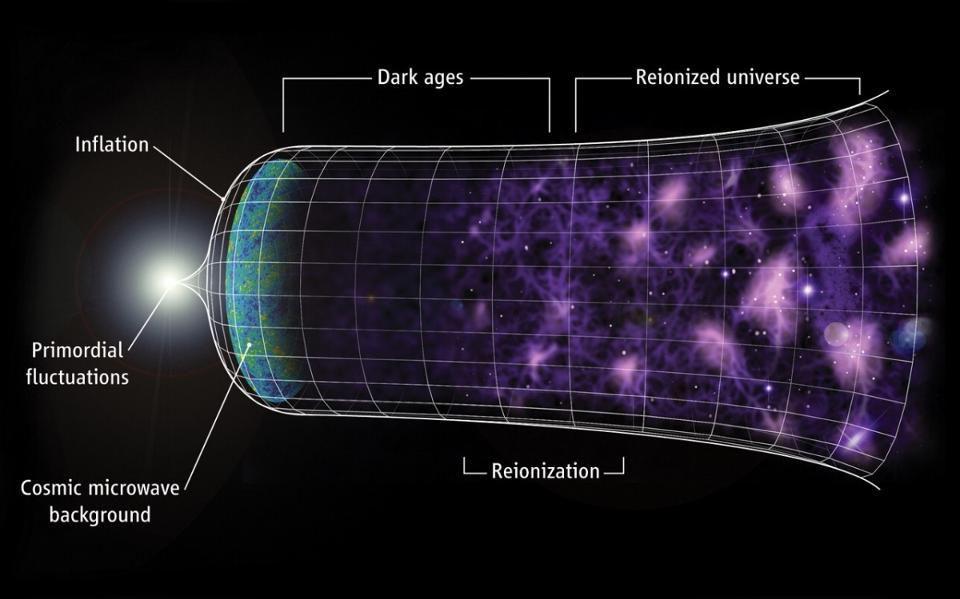
- Studying the Big Bang tells us how our universe evolved to become this way, but it doesn’t immediately reveal why the Big Bang occurred or what might have preceded it.
- Theoretically and observationally, the evidence for cosmic inflation preceding and setting up the Big Bang is incredibly strong and comprehensive.
- There are still some new, sensitive things to measure, but the lack of low-hanging fruit doesn’t mean the tree is dead.
For as long as humans have been around, our innate curiosity has compelled us to ask questions about the universe. Why are things the way they are? How did they get to be this way? Were these outcomes inevitable or could things have turned out differently if we rewound the clock and began things all over again? From subatomic interactions to the grand scale of the cosmos, it’s only natural to wonder about it all. For innumerable generations, these were questions that philosophers, theologians, and mythmakers attempted to answer. While their ideas may have been interesting, they were anything but definitive.
Modern science offers a superior way of approaching these puzzles. For this week’s inquiry, Jerry Kauffman asks about one of the most fundamental puzzles:
“It’s always troubling for me to think of the Big Bang as having happened at a single point in [spacetime]… What existed before the Big Bang? And why did the Big Bang happen?”
When it comes to even the biggest questions of all, science provides us with the best answers we can muster, given what we know and what remains unknown, at any point in time. Here and now, these are the best robust conclusions we can reach.
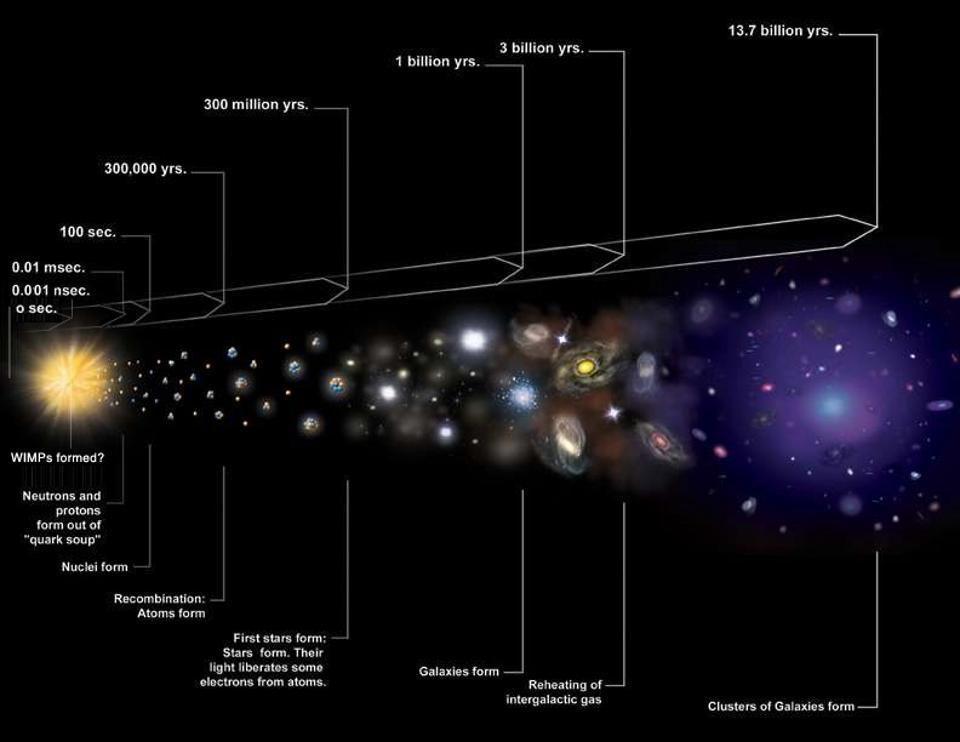
When we look out at the galaxies in the universe today, we find that — on average — the farther away it is, the greater the amount its light is shifted toward longer and redder wavelengths. The longer light spends traveling through the universe before it reaches our eyes, the greater the amount that the expansion of the universe stretches its wavelength; this was how we discovered that the universe is expanding. Because stretched, longer-wavelength light is colder than shorter-wavelength light, the universe cools as it expands. If we extrapolate backward in time instead of forward, we’d expect the early universe to exist in a hotter, denser, more uniform state.
Originally, we took the extrapolation as far back as we could imagine — to infinite temperatures and densities, and an infinitesimally small volume: a singularity. Evolving forward from that initial state, we successfully predicted and later observed:
- the leftover radiation from the Big Bang, observable as the cosmic microwave background
- the abundance of the light elements before any stars were formed
- the gravitational growth of large-scale structure in the universe
However, we also observed things we couldn’t explain if the universe began from a singular state, including why there were no leftover relics from the highest-energy epochs, why the universe had the same properties in opposite directions that could never have exchanged information with one another, and why there was absolutely no spatial curvature, leaving the universe indistinguishable from flat.
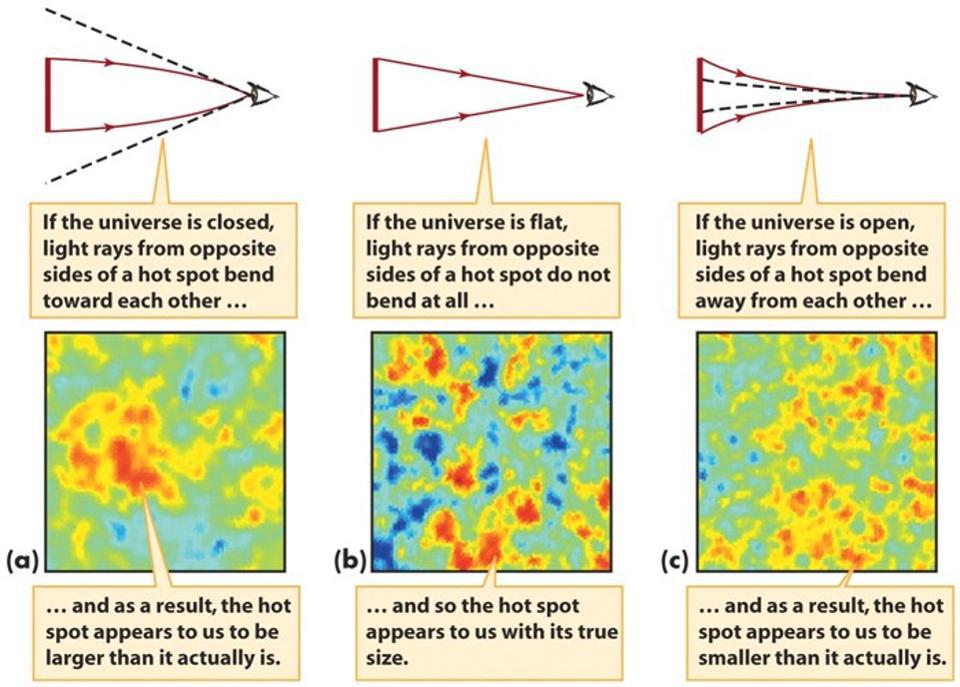
Credit: Smoot Cosmology Group/LBL
Whenever we reach this scenario — observing properties that our leading theories cannot explain or predict — we are left with two options:
- You can pawn off the properties as “initial conditions.” Why is the universe flat? It was born that way. Why is it the same temperature everywhere? Born that way. Why aren’t there high-energy relics? They must not exist. And so on. This option offers no explanation.
- You can imagine some sort of dynamics: a mechanism that precedes the state we’ve observed and sets it up, so that it started off with the conditions necessary to create the properties we observe today.
Although it’s a bit controversial to say, the first option is only acceptable when you are certain that the conditions you could have started off with are sufficiently random. For example, solar systems form from instabilities in protoplanetary disks around newly forming stars; that’s random, and so there’s no explanation for why our solar system possesses its particular set of planets. But for the entire universe, choosing that option is tantamount to giving up on dynamics, asserting that there’s no need to even search for a mechanism that could have preceded and set up the hot Big Bang.
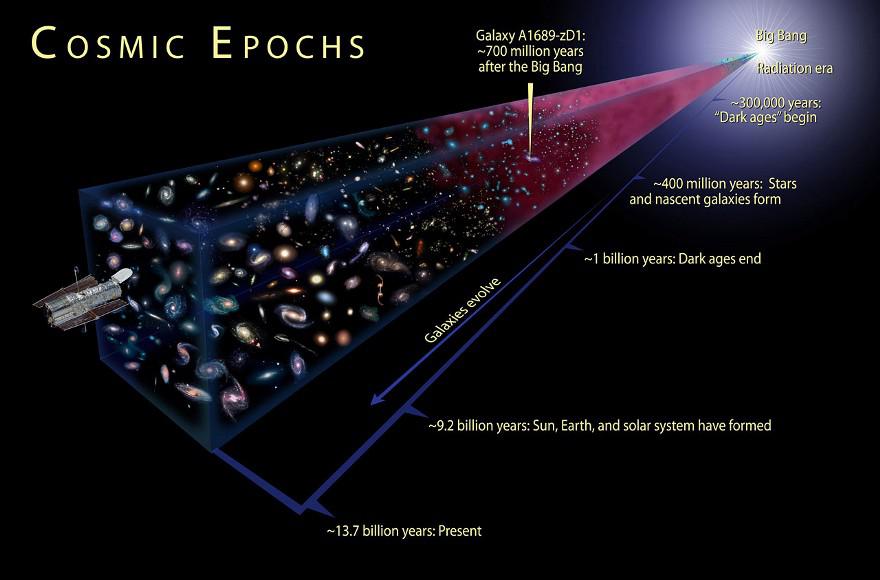
Credit: NASA, ESA, and A. Feild (STScI)
Fortunately, however, not everyone fell into that solipsistic logical fallacy. If you want to go beyond your current understanding of how things work, all it takes is a new, superior idea. How do you know whether an idea is good enough to supersede our old theory and revolutionize our view of the universe? Believe it or not, there are just three criteria you have to meet:
- It has to reproduce every success that the old theory achieved. Every single one, with no exception.
- It has to succeed where the old theory didn’t, by successfully explaining the phenomena the old theory couldn’t.
- It needs, perhaps most importantly, to make novel predictions that differ from the old theory’s predictions. These novel predictions must then be tested to determine the new idea’s failure or success.
That was precisely what, a little more than 40 years ago, the concept of cosmic inflation (sometimes known as cosmological inflation) set out to do. It hypothesized that before the universe was filled with matter and radiation, it was dominated by energy inherent to the fabric of space itself. That energy caused the universe to expand exponentially and relentlessly. The expansion would stretch space so that it was seemingly flat, causing all directions to have the same temperature because everything was causally connected in the past. Ultimately, this process would place an upper limit on the maximum temperature achieved in the early universe, preventing the formation of high-energy relics.
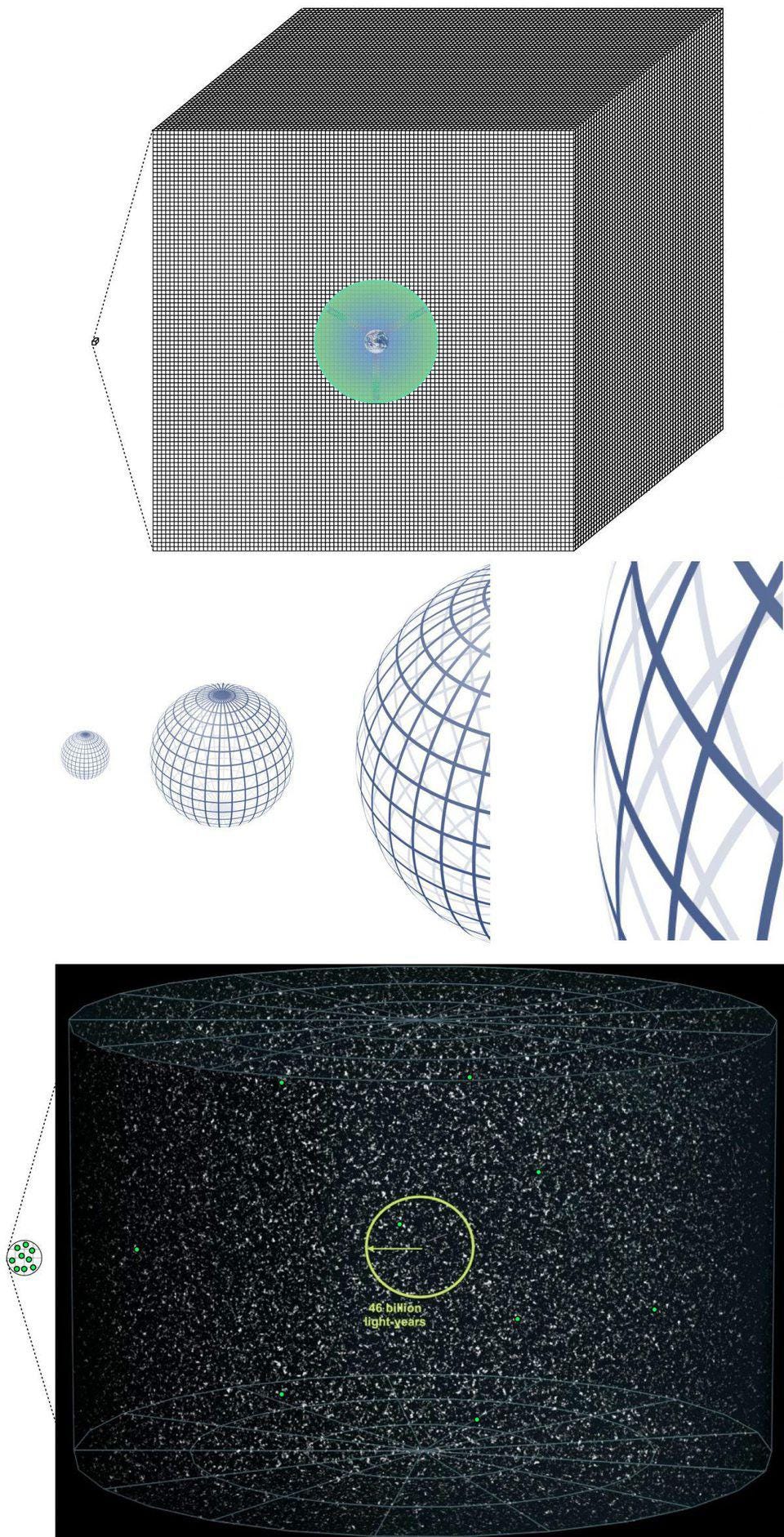
Credit: E. Siegel/Beyond the Galaxy
The initial model of cosmic inflation succeeded where the Big Bang without inflation failed, but it struggled to meet the first criterion, in that it failed to produce a universe that had uniform properties in all directions. However, with the work of the community, class models were swiftly discovered that reproduced the Big Bang’s successes, and that led to a rich era of theoretical exploration. We would model cosmic inflation as a field, and then the laws of physics would enable us to extract the properties imprinted on the universe from any particular model we chose. These details were worked out largely during the 1980s and the 1990s, and are found in a variety of textbooks in the field, including:
- Kolb and Turner’s The Early Universe
- John Peacock’s Cosmological Physics
- Liddle and Lyth’s Cosmological Inflation and Large-Scale Structure
- Scott Dodelson’s Modern Cosmology
Dodelson’s book became the field’s standard on how cosmic inflation’s imprints are left on the universe, particularly in the cosmic microwave background. If you studied cosmology at the graduate level within the past 30 years, these were many of the seminal primary sources that taught you how to extract some key predictions from inflation that would differ from a universe where inflation did not occur.
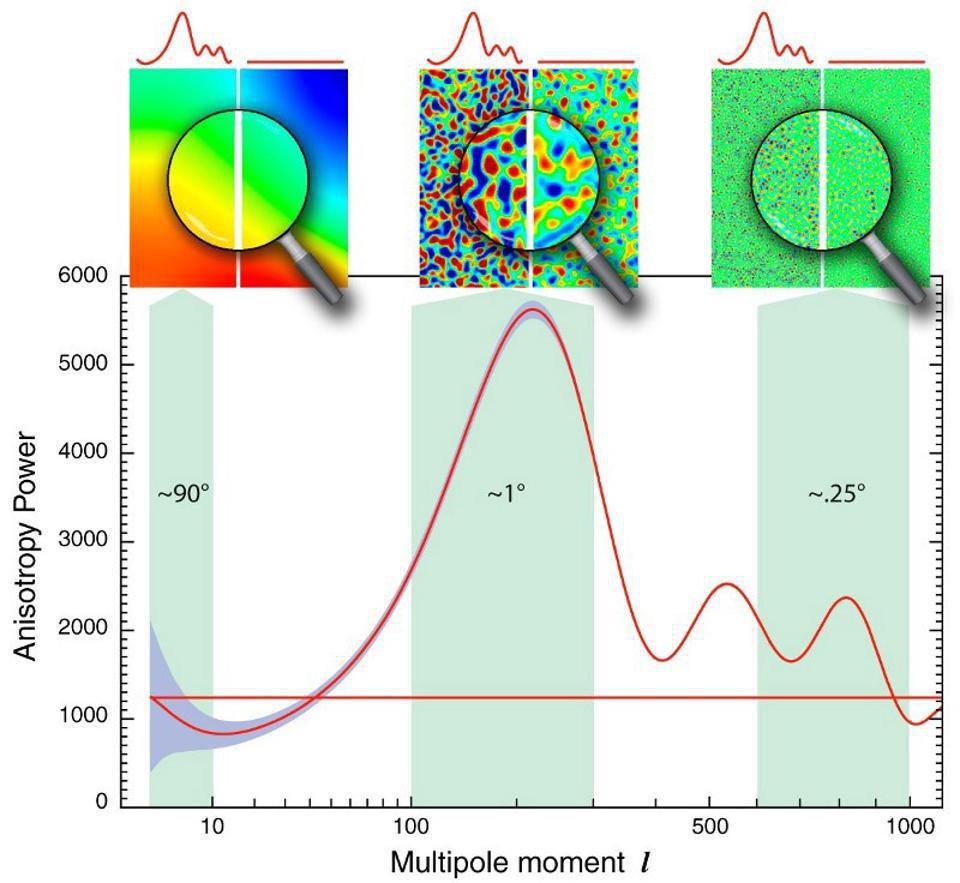
Credit: NASA/WMAP Science Team
In particular, there are six major predictions of cosmic inflation that were definitively extracted before they were ever put to the test. Inflation predicts:
- a spectrum of imperfections — density and temperature fluctuations — that are almost, but not perfectly, scale-invariant
- a universe that’s coarsely indistinguishable from flat, but that has curvature to it at the ~0.001% level
- density imperfections that are 100% adiabatic and 0% isocurvature in nature
- fluctuations on super-horizon scales, which are larger than a signal moving at the speed of light in an expanding universe could create
- a finite maximum temperature to the universe during the hot Big Bang, which should be significantly smaller than the Planck scale
- a spectrum of gravitational wave fluctuations — tensor fluctuations — should be created as well, with a particular pattern to it.
All six of these predictions were in place long before the first data from the WMAP or Planck satellites came back, allowing us to test cosmic inflation versus a non-inflationary scenario. We’ve since observed strong evidence favoring cosmic inflation for points 1, 3, 4, and 5, and have yet to reach sensitivities that reveal a decisive signal for points 2 and 6. However, going 4-for-4 where we’ve been able to test it has been more than sufficient to validate inflation, rendering it the new consensus explanation for the origin of our universe. Inflation came before and set up the hot Big Bang, with extrapolation back to a singularity having now become an unfounded assumption.

Credit: Nicole Rager Fuller/National Science Foundation
A little deeper
However, as is almost always the case in science, learning something new about the universe only raises additional questions. What exactly is the nature of cosmic inflation? How long was its duration? What caused the universe to inflate at all? If cosmic inflation is caused by a quantum field — a justifiable assumption to make — then what are the properties of that field? Just as before, if we want to answer these questions, we have to find ways of testing the nature of inflation and then subject the universe to those tests.
The way we explore this is by building inflationary models — leveraging effective field theories — and extracting the key predictions from various models of inflation. Generically, you have a potential, you get inflation when the ball is “high up on a hill” on the potential, and inflation ends when the ball rolls down from a high point into a “valley” of the potential: a minimum. By calculating various properties of cosmic inflation from these potentials, you can extract predictions for the signals you expect to exist in your universe.
Then, we can go out and measure the universe, such as by measuring some precise and intricate properties of the light that composes the cosmic microwave background, and compare them to the various models we’ve concocted. The ones that remain consistent with the data are still viable, while the ones in conflict with the data can be ruled out. This interplay of theory and observation are how all astronomical sciences, including cosmology and the science of the early universe, advance.
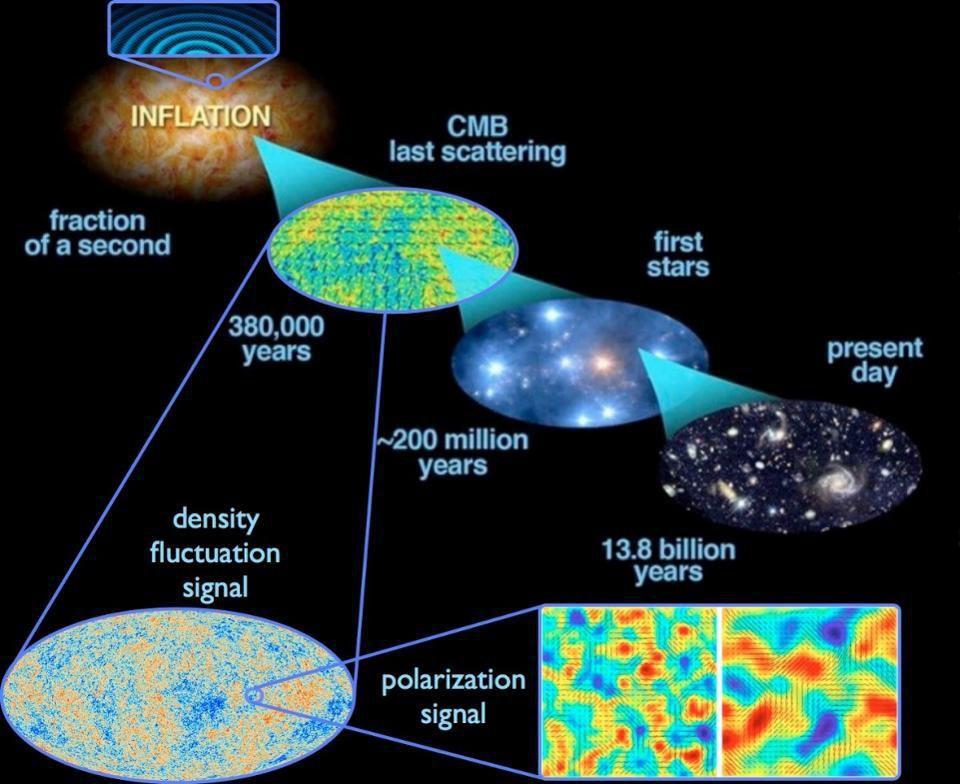
Credit: E. Siegel; ESA/Planck and the DOE/NASA/NSF Interagency Task Force on CMB research
In all inflationary models, it’s the final moments of cosmic inflation — the ones that occur just prior to the onset of the hot Big Bang — that leave their imprints on the universe. These final moments always produce two types of fluctuations:
- scalar fluctuations. These appear as density/temperature imperfections and lead to the large-scale structure of the universe
- tensor fluctuations. These show up as gravitational waves left over from inflation, and imprint themselves on the polarization of the light from the cosmic microwave background. Specifically, they appear as what we call B-modes: a special type of polarization that happens when light and gravitational waves interact.
How do we determine what the scalar fluctuations and the tensor fluctuations are? As detailed in the aforementioned texts, there are only a few aspects of the inflationary potential that matter. Inflation occurs when you’re high up on the “hill” of a potential inflation ends when you roll into the “valley” below and stay there. The specific shape of the potential, including its first and second derivatives, determine the values of these fluctuations, while the height of the “high point” versus the “low point” of the potential determines what we call r: the ratios of tensor-to-scalar fluctuations. This measurable quantity, r, can be large — up to ~1. But it can also be very small: down to 10-20 or lower without any difficulties.

Credit: Planck Science Team
On the surface, it might seem that cosmic inflation doesn’t predict anything on this front, considering that such widely disparate predictions are possible. For the amplitude of the tensor-to-scalar ratio, r, that’s correct, though each model will have its own unique prediction for r. However, there is a very clean and universal prediction that we can extract: what the spectrum of gravitational wave (tensor) fluctuations should look like, and what their magnitude is on any scale we can examine. When we look at the signals that get imprinted on the cosmic microwave background, we can robustly predict what the relative size of these fluctuations are from small angular scales up to large ones. The only thing that’s unconstrained, except by observation, is the absolute “height” of the spectrum, and hence, the magnitude of r.
In the mid-2000s, there was an NASA/NSF/DOE interagency task force that set about planning a new generation of experiments to measure the polarization of the light from the cosmic microwave background on small angular scales, specifically designed to constrain r and either validate or rule out various models of inflation. Numerous observatories and experiments were designed and built to achieve that goal: BICEP, POLARBEAR, SPTpol, and ACTPOL, to name a few. The goal was to constrain r down to about ~0.001. If the gravitational waves from inflation made a large enough signal, we’d see them. If not, we’d place meaningful constraints and rule out whole classes of inflationary models. With new observational data coming, theorists set about making models with large r values, which would fall in the testing area and hence would be relevant for these experiments.
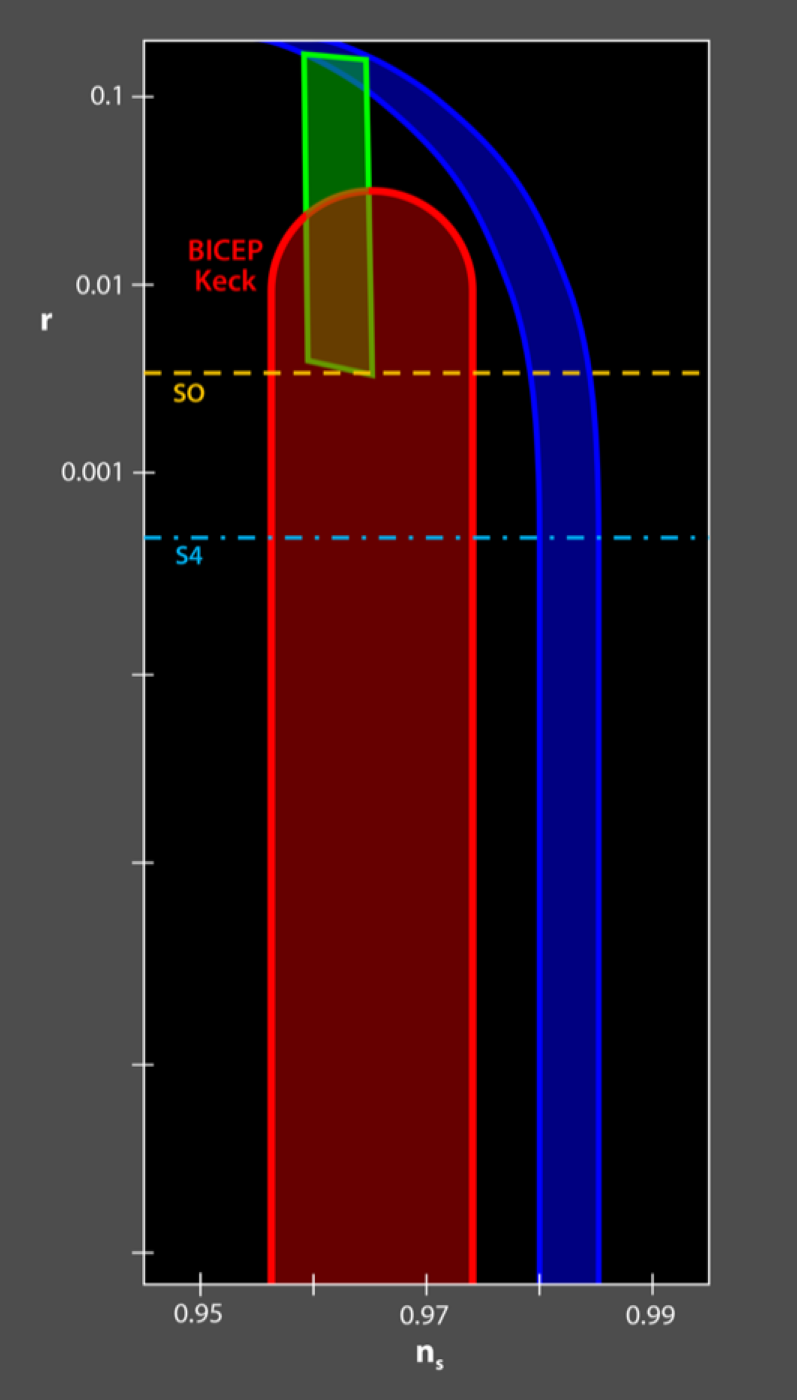
Credit: APS/Alan Stonebreaker, modified by E. Siegel
In many ways, the best data currently comes from the BICEP collaboration, currently on the third iteration of their experiment. There are only upper limits on r, now constrained to be no greater than about 0.03 or so. However, absence of evidence is not evidence of absence. The fact that we haven’t measured this signal doesn’t mean it isn’t there, but rather that if it is there, then it’s below our current observational capabilities.
What failing to find these tensor fluctuations (yet) definitely, definitely does not mean is that cosmic inflation is wrong. Inflation is well-validated by numerous independent observational tests, and would only be falsified by the data if we did detect these tensor modes, and they didn’t follow the precise spectrum predicted by inflation.
And yet, you’d never know any of this by listening to the scientists associated with BICEP and the public-facing communication they’ve put out into the world. They continue to assert that:
- inflation remains in doubt
- B-modes (indicating tensor fluctuations) are necessary to validate inflation
- if there aren’t large magnitude ones, inflation is falsified
- we are likely on the cusp of a paradigm shift
- cyclic models are a viable competitor to inflation
- inflation simply moved the “singular Big Bang” to before inflation, rather than immediately preceding the hot Big Bang
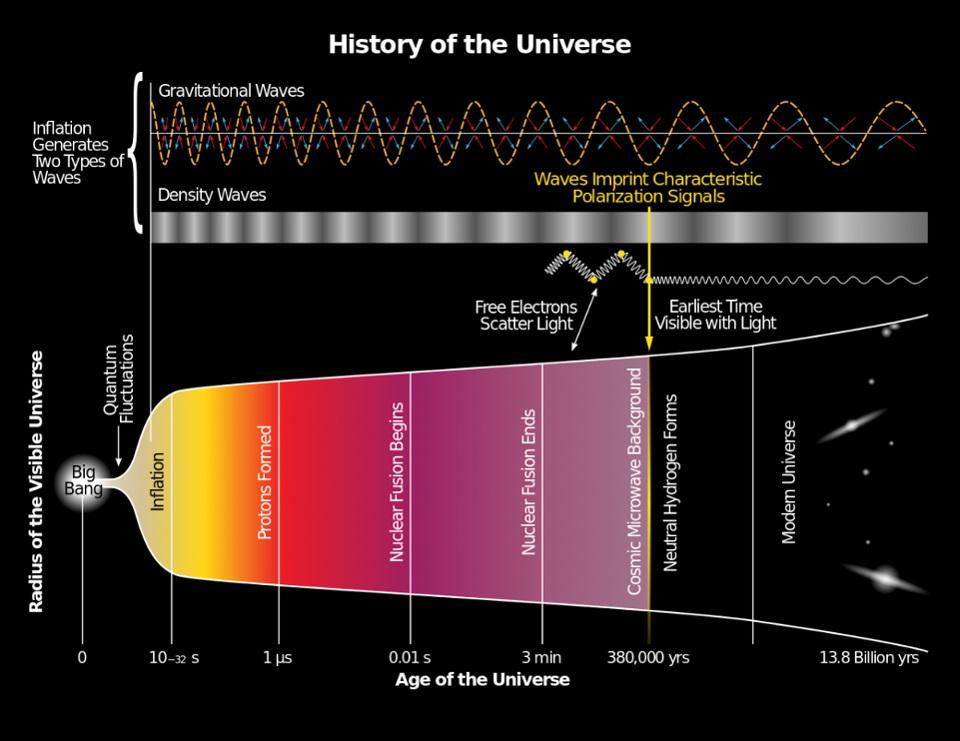
Credit: NSF (NASA, JPL, Keck Foundation, Moore Foundation, Related) – Funded BICEP2 Program
All of these assertions, to be blunt, are both incorrect and irresponsible. Worst of all, every single one of the scientists I’ve spoken to who has made these claims knows they’re incorrect. However, the claims are still advanced — including to the general public through popular treatments — by the very scientists who are running these experiments. There’s no kind way to couch it: if it isn’t self-deception, it’s utter intellectual dishonesty. In fact, when a scientist makes an overblown and premature claim that turns out, on closer inspection, to be completely wrong, some of us in the astronomical community call that a “BICEP2,” named after the infamous false discovery they announced back in 2014.
Most of all, it’s a pity. These experiments that measure the properties of the cosmic microwave background to such extraordinary precisions are giving us the best information we’ve ever had about the nature of the universe, and of the inflationary epoch that preceded and set up — and caused — the hot Big Bang. Cosmic inflation is well validated as the origin of our universe. It has replaced the noninflationary, singularity-containing Big Bang as our cosmological standard model for where we all came from. Although there are contrarian alternatives out there, none of them have ever succeeded where cosmic inflation does not. Meanwhile, they all fail to reproduce the full suite of inflation’s successes.
Scientists who value glory and attention over accuracy will no doubt continue to make baseless assertions undercutting what’s actually known about the universe. But don’t be fooled by such claims. At the end of the day, we learn what exists in the universe by asking it questions about itself and listening to its response. As soon as we abandon that approach, we have to admit the uncomfortable truth: we simply aren’t doing science anymore.
Send in your Ask Ethan questions to startswithabang at gmail dot com!