This Is Why Neutrinos Are The Standard Model’s Greatest Puzzle
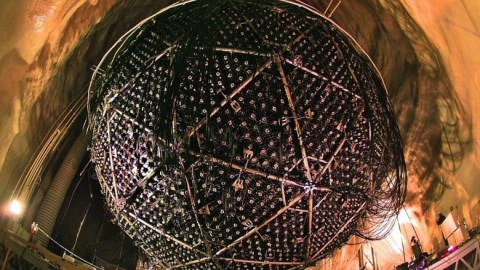
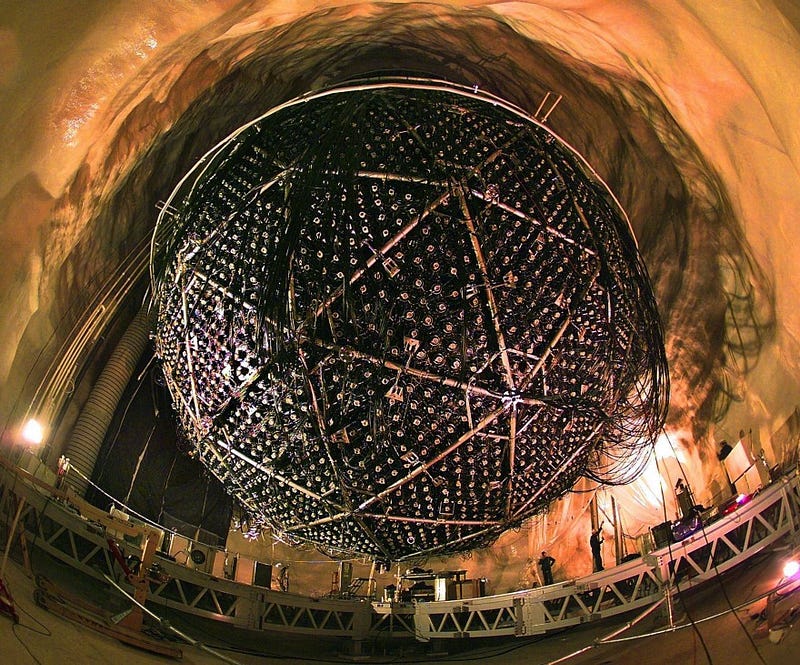
No other particles behave the way the elusive neutrino does, and that might unlock our greatest mysteries.
Every form of matter that we know of in the Universe is made up of the same few fundamental particles: the quarks, leptons and bosons of the Standard Model. Quarks and leptons bind together to form protons and neutrons, heavy elements, atoms, molecules, and all the visible matter we know of. The bosons are responsible for the forces between all particles, and — with the exception of a few puzzles like dark matter, dark energy, and why our Universe is filled with matter and not antimatter — the rules governing these particles explains everything we’ve ever observed.
Except, that is, for the neutrino. This one particle behaves so bizarrely and uniquely, distinct from all the others, that it’s the only Standard Model particle whose properties cannot be accounted for by the Standard Model alone. Here’s why.
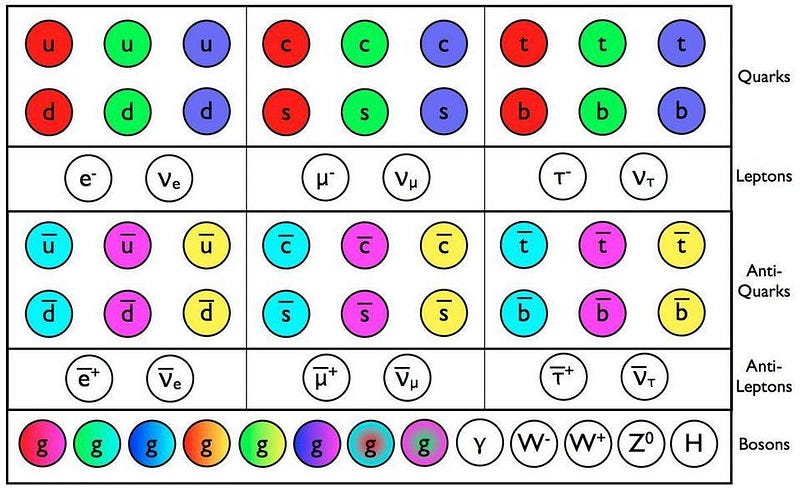
Imagine you have a particle. It’s going to have a few specific properties that are intrinsically, unambiguously known. These properties include:
- mass,
- electric charge,
- weak hypercharge,
- spin (inherent angular momentum),
- color charge,
- baryon number,
- lepton number,
- and lepton family number,
as well as others. For a charged lepton, like an electron, values like mass and electric charge are known to an extraordinary precision, and those values are identical for every electron in the Universe.
Electrons, like all quarks and leptons, also have values for all of these other properties (or quantum numbers). Some of those values may be zero (such as color charge or baryon number), but the non-zero ones tell us something additional about each particle in question. Spin, for example, can be either +½ or -½ for the electron, which tells you something important: there’s a degree of freedom here.

It’s the reason why, if you bind an electron to a proton (or any atomic nucleus), there’s a 50/50 shot that the electron will have its spin aligned with the proton’s spin, and a 50/50 shot that they’ll be anti-aligned. An electron’s spin, relative to any axis you choose (x, y, and z, the electron’s direction of motion, the proton’s spin axis, etc.) is completely random.
Neutrinos, like electrons, are also leptons. Although they don’t have electric charge, they do have quantum numbers all their own. Just as an electron has an antimatter counterpart (the positron), the neutrino has an antimatter counterpart as well: the antineutrino. Although they were first theorized in 1930 by Wolfgang Pauli, the first neutrino detection didn’t take place until the mid-1950s, and actually involved antineutrinos produced by nuclear reactors.
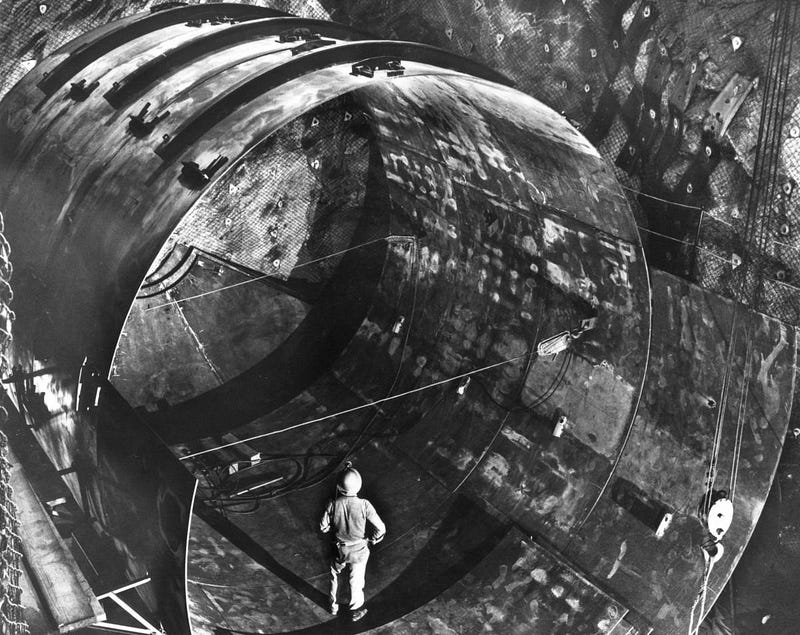
Based on the properties of the particles produced by a neutrino interaction, we can reconstruct various properties of the neutrinos and antineutrinos that we see. One of them, in particular, stands out as incongruent with every other fermion in the Standard Model: spin.
Remember how there was a 50/50 shot that an electrons would have a spin of either +½ or -½? Well, that’s true for every quark and lepton in the Standard Model, except the neutrino.
- All six of the quarks and all six of the antiquarks can have spins that are either +½ or -½, with no exceptions.
- The electron, muon, and tau, as well as their antiparticles, are allowed spins of either +½ or -½, with no exceptions.
- But when it comes to the three types of neutrinos and the three types of antineutrinos, their spins are restricted.
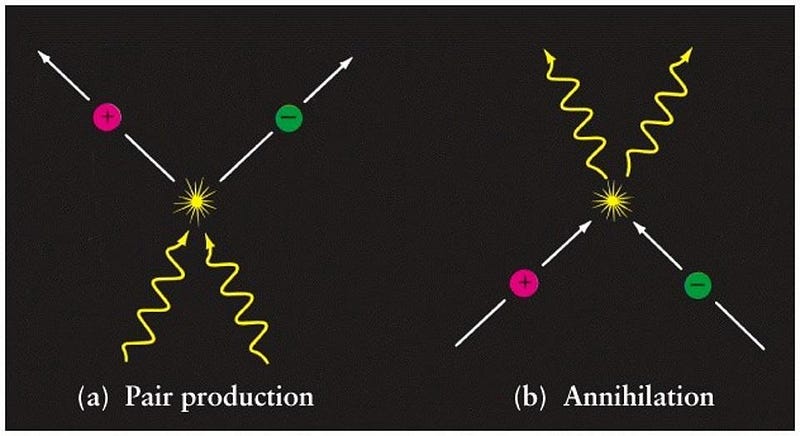
There’s a good reason for this. Imagine you produce a matter/antimatter pair of particles. We’ll imagine three cases: one where the pair is of electrons and positrons, a second where the pair is of two photons (bosons that are their own antiparticle), and a third where the pair is a neutrino and an antineutrino. Starting at the creation point, where the particles first come into existence from some form of energy (via Einstein’s famous E = mc2), you can imagine what will happen for each of these cases.
1.) If you produce electrons and positrons, they’ll move away from one another in opposite directions, and both the electron and positron will have the options of spin being either +½ or -½ along any axis. So long as the total amount of angular momentum is conserved for the system, there are no restrictions on the directions in which electrons or positrons spin.
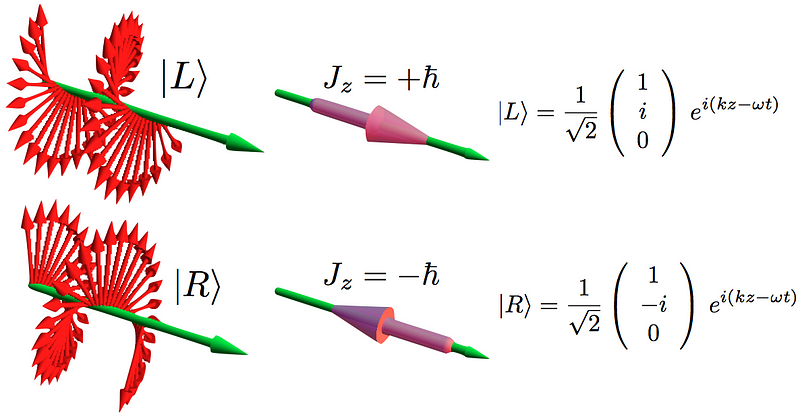
2.) If you produce two photons, they’ll also move away from one another in opposite directions, but their spins are highly constrained. Whereas an electron or positron could spin in any direction at all, a photon’s spin can only be oriented along the axis that this quantum of radiation propagates. You can imagine pointing your thumb in the direction the photon moves, but the spin is restricted by the direction your fingers curl around relative to your thumb: it can go clockwise (right-handed) or counterclockwise (left-handed) around the axis of rotation (+1 or -1; bosons have integer, rather than half-integer, spins), but no other spins are allowed.
3.) Now, we come to the neutrino and antineutrino pair, and it’s going to get weird. All of the neutrinos and antineutrinos we’ve ever detected are extraordinarily high in energy, meaning that they move at speeds so high that their motion is experimentally indistinguishable from the speed of light. Instead of behaving like electrons and positrons, we find that all neutrinos are left-handed (spin = +½) and all antineutrinos are right-handed (spin = -½).
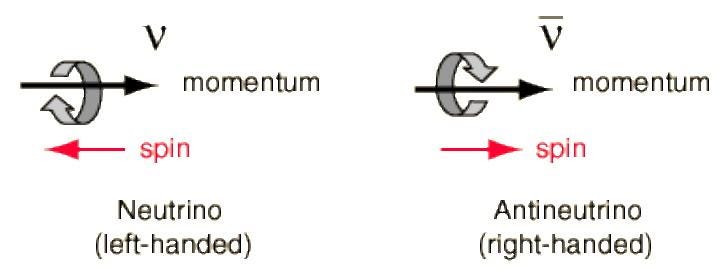
Throughout most of the 20th century, it was taken as an unusual but quirky property of neutrinos: one that was allowed because they were thought to be completely massless. But a series of experiments and observatories involving neutrinos produced by the Sun and neutrinos produced by cosmic ray collisions with Earth’s atmosphere revealed a bizarre property of these elusive particles.
Instead of remaining the same flavor of neutrino or antineutrino (electron, muon, and tau; one corresponding to each of the three families of lepton), there is a finite probability that one type of neutrino can oscillate into another. The probability of this occurring depends on a number of factors that are still being explored, but one thing is certain: this behavior is only possible if neutrinos have a mass. It may be small, but it must be non-zero.
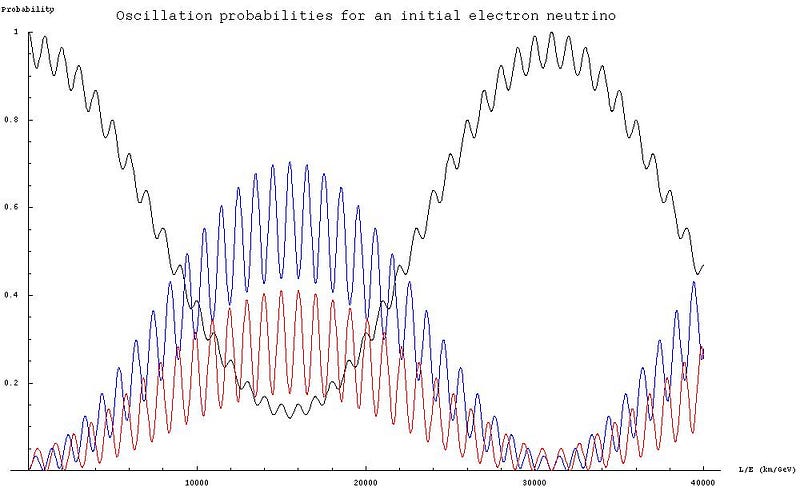
Although we don’t know which neutrino types have which mass, there are meaningful constraints that teach us profound truths about the Universe. From the neutrino oscillation data, we can determine that at least one of these three neutrinos has a mass that can be no less than a few hundredths of an electron-volt; that’s a lower limit.
On the other hand, brand new results from the KATRIN experiment constrain the electron neutrino’s mass to be less than 1.0 eV (directly), while astrophysical data from the cosmic microwave background and baryon acoustic oscillations constrain the sum of the masses of all three types of neutrino to be less than about 0.17 eV. Somewhere between these upper limits and the oscillation-informed lower limit lies the actual masses of the neutrinos.
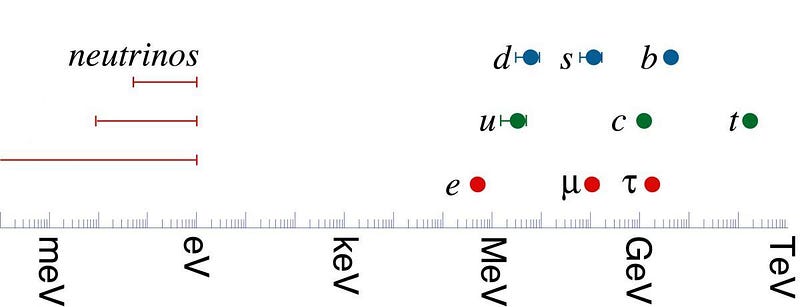
But this is where the big puzzle comes in: if neutrinos and antineutrinos have mass, then it should be possible to turn a left-handed neutrino into a right-handed particle simply by either slowing the neutrino down or speeding yourself up. If you curl your fingers around your left thumb and point your thumb towards you, your fingers curl clockwise around your thumb. If you point your left thumb away from you, though, your fingers appear to curl counterclockwise instead.
In other words, we can change the perceived spin of a neutrino or antineutrino simply by changing our motion relative to it. Since all neutrinos are left-handed and all antineutrinos are right-handed, does this mean that you can transform a left-handed neutrino into a right-handed antineutrino simply by changing your perspective? Or does this mean that left-handed antineutrinos and right-handed neutrinos exist, but are beyond our current detection capabilities?
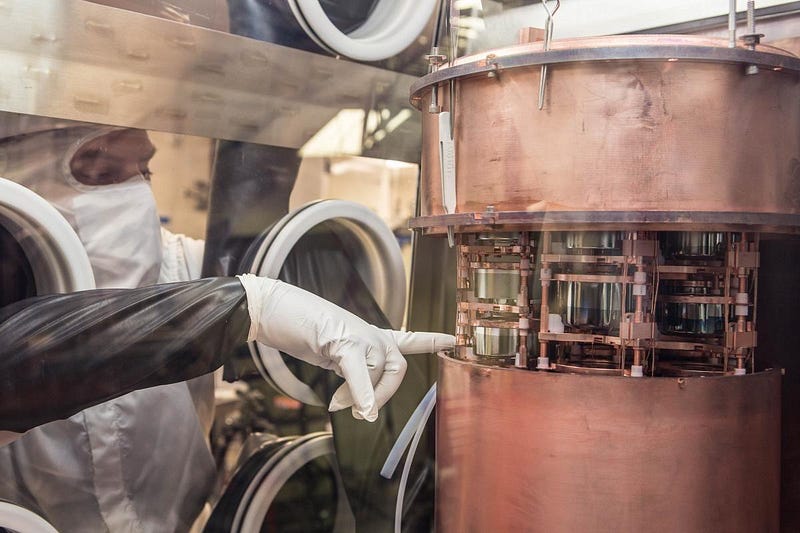
Believe it or not, unlocking the answer to this question could open the door to understanding why our Universe is made of matter and not antimatter. One of the four fundamental requirements for producing a matter-antimatter asymmetry from an initially symmetric state is for the Universe to behave differently if you replace all the particles with antiparticles, and a Universe where all your neutrinos are left-handed and all your antineutrinos are right-handed could give you exactly that.
The result of boosting yourself to view a left-handed neutrino from the opposite direction will shed a tremendous hint: if you see a right-handed neutrino, then they exist in this Universe, neutrinos are Dirac fermions, and there’s something more to learn. If you see a right-handed antineutrino, however, then neutrinos are Majorana fermions, and might point towards a solution (leptogenesis) to the matter-antimatter problem.
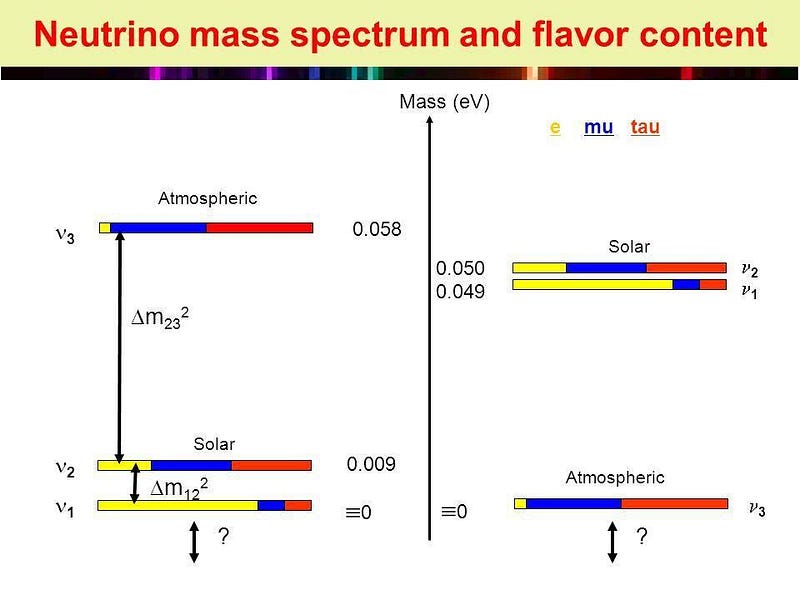
Our Universe, as we understand it today, is full of puzzles that we cannot explain. The neutrino is perhaps the only Standard Model particle whose properties have yet to be thoroughly uncovered, but there’s a tremendous hope here. You see, during the earliest stages of the Big Bang, neutrinos and antineutrinos are produced in tremendous numbers. Even today, only photons are more abundant. On average, there are around 300 neutrinos and antineutrinos per cubic centimeter in our Universe.
But the ones that were made in the Universe’s hot, early stages are special: as a result of being around for so long in our expanding Universe, they now move so slowly that they’re guaranteed to have fallen into a large halo encompassing every massive galaxy, including our own. These neutrinos and antineutrinos are everywhere, with minute but finite cross-sections, just waiting to be explored. When our experimental sensitivity catches up to the physical reality of relic neutrinos, we’ll be one step closer to understanding just how, exactly, our Universe came to be. Until then, neutrinos will likely remain the Standard Model’s greatest puzzle.
Ethan Siegel is the author of Beyond the Galaxy and Treknology. You can pre-order his third book, currently in development: the Encyclopaedia Cosmologica.