This Is How Our Earliest Picture Of The Universe Shows Us Dark Matter

If you go all the way back to where neutral atoms first formed, you can see the cosmic microwave background. Buried in the details is the Universe’s first evidence for dark matter.
One of the greatest mysteries of modern science is the puzzle of dark matter. If you add up all the normal matter making up planets, stars, gas, plasma, black holes, galaxies, and the space between galaxies — all the matter in the known Universe — it isn’t enough to explain the gravity we see. It can’t explain individual galaxies, clusters of galaxies, colliding groups of galaxies, gravitational lensing, or the large-scale structure of the Universe. Something more must be out there, and it can’t be normal matter.
The name we’ve given this mysterious substance is dark matter. Dark because it doesn’t interact with light or normal matter; it can’t be seen. Matter because it gravitates, clumps, and clusters together. Although there’s a controversy over exactly what dark matter is, its existence is virtually certain, as it shows up in every astronomical observation possible. Even, as we discovered earlier this century, in the earliest picture of the Universe we could ever take: of the Big Bang’s leftover glow.
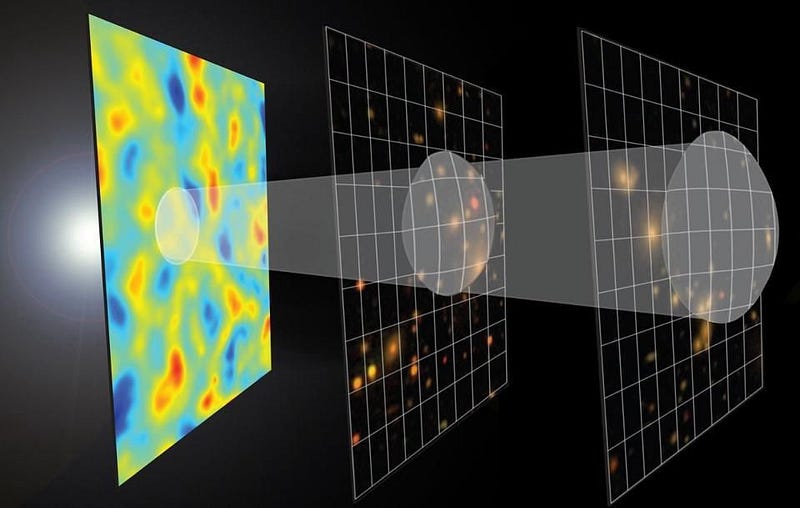
Billions of years ago, closer back in time to the Big Bang, the Universe was denser and more uniform. It takes billions of years to form the large galaxy clusters we see today, hundreds of millions to form the first galaxies, and tens of millions to form the first stars. Because an expanding Universe also cools — the energy of any individual photon is proportional to its wavelength, and all “lengths” stretch (to lower energies) as the Universe expands — the early Universe was not just smaller, but also hotter. At some point in the past, the Universe was hot enough that every neutral atom that formed, every electron bound to an atomic nucleus, would be dissociated into free ions by the radiation that was created in the hot Big Bang.
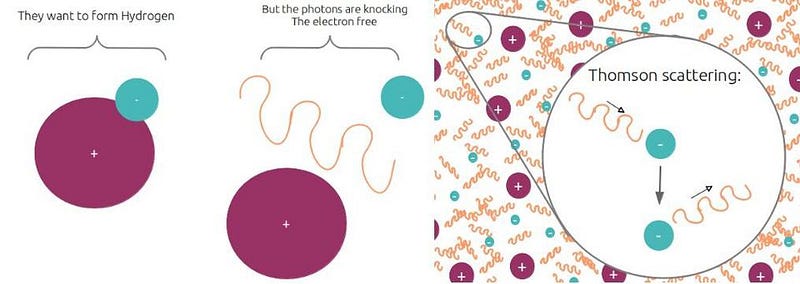
Before it was cool enough to stably form neutral atoms, you had photons flying around, smacking into electrons willy-nilly. It was happening all the time, everywhere you went. After you form neutral atoms, only photons of a very, very particular wavelength — the wavelengths that result in either ionization or atomic transitions of that particular atom — can interact. Prior to the Universe cooling through this threshold, photons and normal matter interact at an extremely high rate. After the Universe cools through this threshold, i.e., after the Universe becomes 100% filled with neutral atoms and 0% filled with ions, those photons just stream in a straight line. Their wavelength, over the past 13.8 billion years, stretches as the Universe expands. And finally, today, it arrives at our eyes and our detectors.
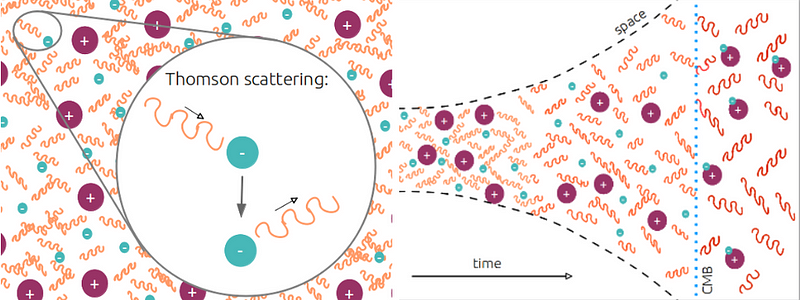
Originally, we had a great word for this leftover radiation from the Big Bang: the primeval fireball. Once we discovered it in the mid-1960s, however, we learned what its temperature and wavelength/frequency properties were: it existed at 2.725 K, placing it in the microwave portion of the spectrum. It had the same temperature properties in all directions on the sky, and became known as the Cosmic Microwave Background (CMB) radiation. For a long time, the “uniform temperature” was the defining characteristic of the CMB. The only imperfections we saw arose from other matter that absorbed, emitted, or modified microwave radiation, such as the galactic plane of the Milky Way.
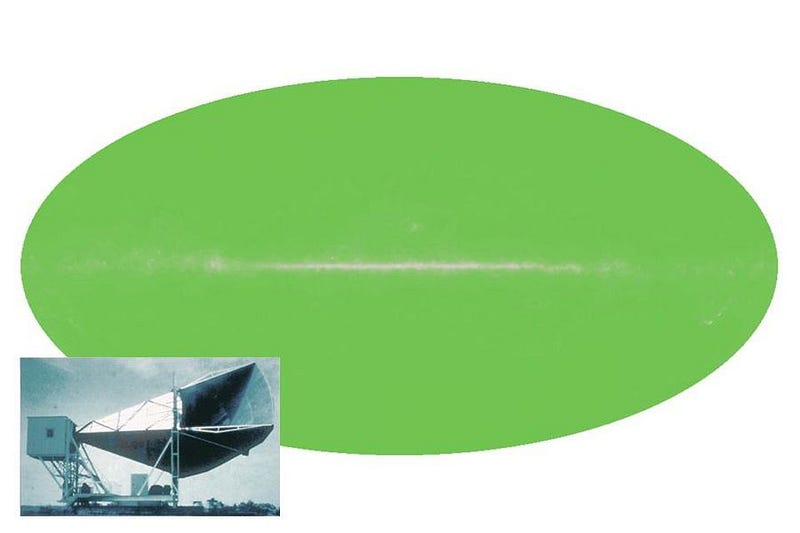
But as our satellites and balloon-borne experiments became better, we started to see the cosmic imperfections in the CMB. These are vitally important: without overdense and underdense regions, there’s no way to grow structure like stars, galaxies, and clusters of galaxies. The scales and magnitudes of these initial fluctuations determine what our Universe, today, will wind up looking like. The fact that we have the massive, diverse cosmic structures we have today are a testament to just how important these seed fluctuations are.
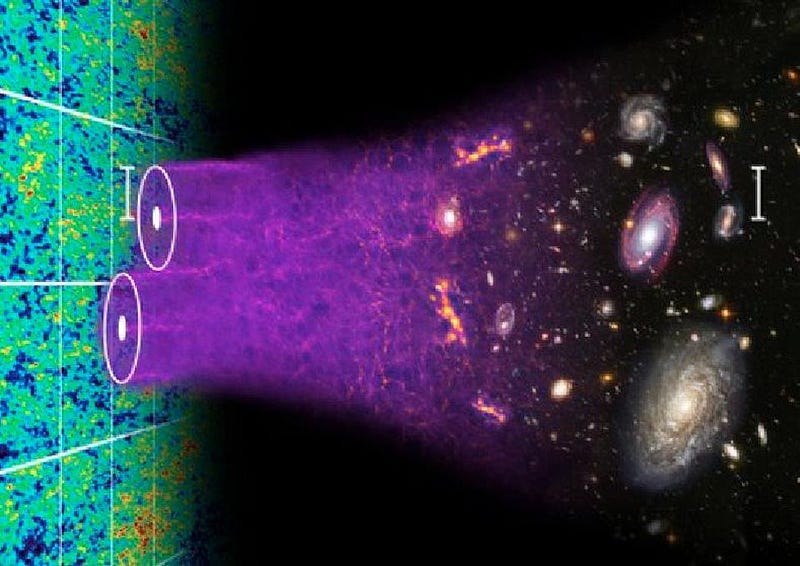
In the 1990s, we sent up the COBE satellite, and measured the fluctuations on the largest of scales, finding that they existed at the ~0.003% level. In the 2000s, WMAP brought us down to smaller angular scales of about a single degree, and then Planck in the 2010s brought us down to just 0.07 degrees: the smallest scale yet. While it might not be obvious, these fluctuations don’t just tell us what the Universe will evolve into as we move forward, providing the seeds of large-scale structure, but they also allow us to figure out what, exactly, the Universe is made of.
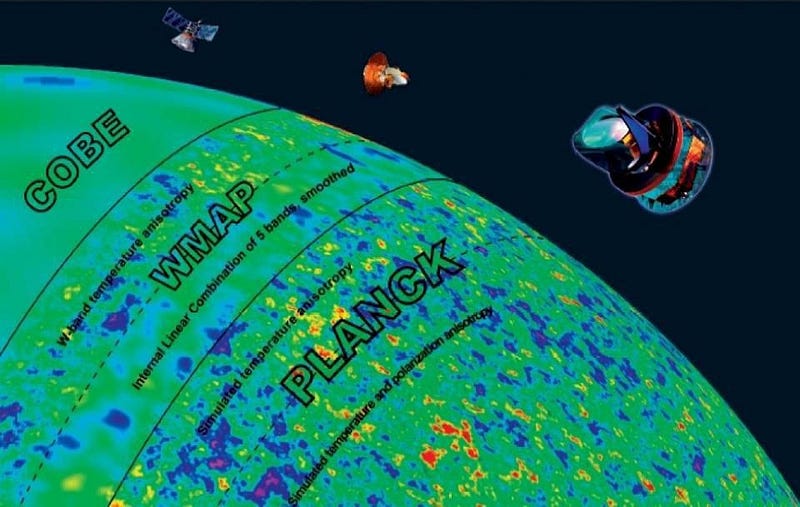
There ought to be density fluctuations that the Universe is “created” with: these are cosmic imperfections imprinted on the Universe, on all scales, from the end of cosmic inflation. They show up on all scales from the moment of the Big Bang, providing these overdense and underdense regions.
Over time, however, the Universe doesn’t just expand and cool, but the overdense regions try to grow, attracting more matter based on what’s in the Universe. The underdense regions fail at growing, and attempt to give up their matter to the less dense regions surrounding them. But this can’t proceed because of one pesky problem: the normal matter in the Universe and the photons (radiation) in the Universe interact with one another, smacking into each other, until those neutral atoms form.
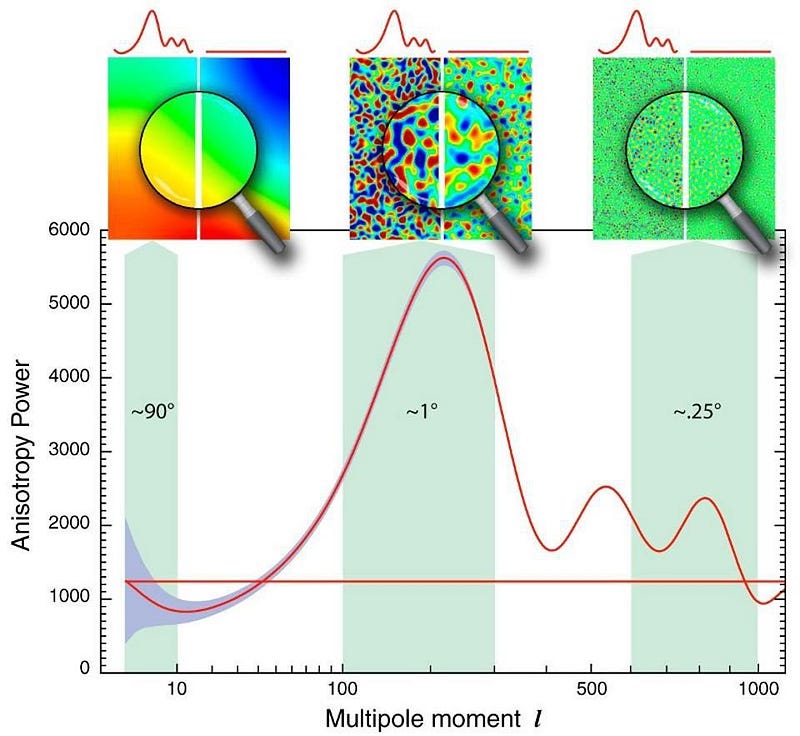
In a Universe with just normal matter and radiation, gravitation tries to pull the normal matter into denser regions, but the radiation works against it. Create an overdense region, and that causes the radiation pressure inside to rise, which — and this is key — pushes out against the normal matter. As much time has passed since the Big Bang determines how far the radiation can travel, and therefore, on what scales it can push that normal matter out.
But if there’s dark matter in the Universe, something extra happens. Yes, it gravitates, and yes, the growing overdensities cause the radiation pressure to increase in the corresponding locations. But there’s no direct interaction cross-section between normal matter and dark matter, nor between radiation and dark matter. As a result, the pattern of peaks-and-valleys that will arise in the CMB will be different depending on how much of each ingredient is in your Universe.
Most dramatically, you can simulate what a Universe that has no dark matter will look like, and what a Universe with the amount of dark matter we think we have — 5 times the amount of normal matter — from large-scale structure and X-ray cluster observations. If you start these two sample Universes off shortly after the Big Bang and just let them evolve, they both create peaks-and-valleys in the CMB as the normal matter and photons dance, but the dark matter both changes the overall matter-radiation dance and also adds a different dance atop it.
Below, to the left (with dark matter) and to the right (without dark matter), you can see the results.
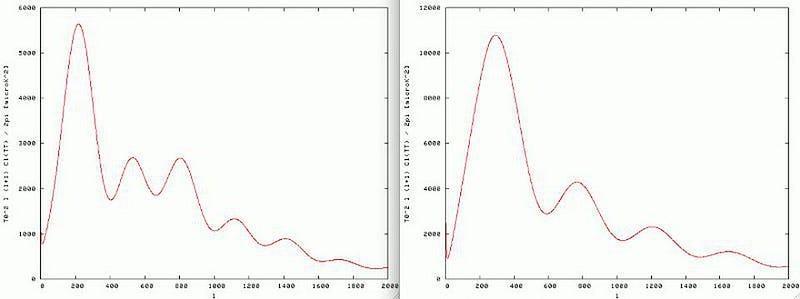
So all you need to do, to know whether your Universe has dark matter or not, is to measure these temperature fluctuations that appear in the CMB! The relative heights, locations, and numbers of the peaks that you see are caused by the relative abundances of dark matter, normal matter, and dark energy, as well as the expansion rate of the Universe. Quite importantly, if there is no dark matter, you only see half as many total peaks! When we compare the theoretical models with the observations, there’s an extremely compelling match to a Universe with dark matter, effectively ruling out a Universe without it.
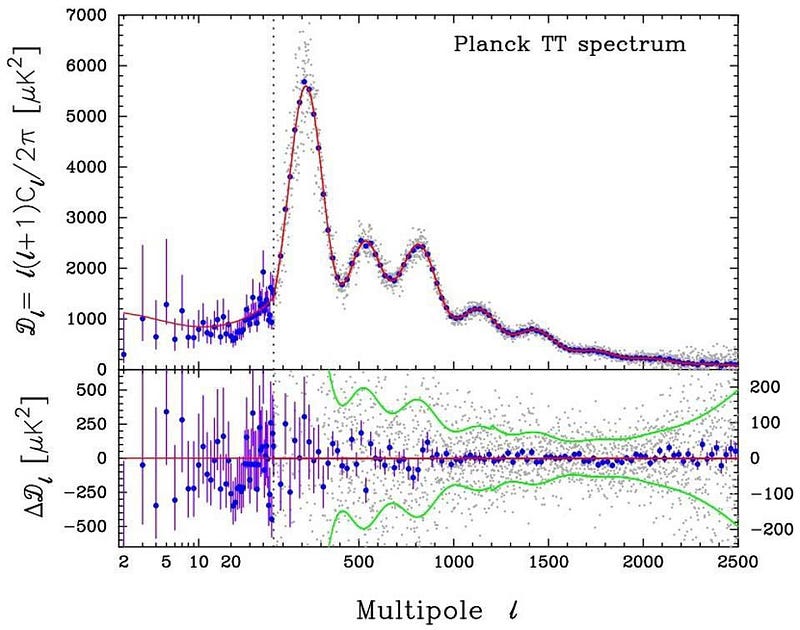
The mere fact that there are as many peaks in the CMB as there are tells us there must be dark matter. The ratios of the peak heights, and the measurement of the Hubble constant to be around 70 km/s/Mpc, tells us that the Universe is roughly 68% dark energy, 27% dark matter, 5% normal matter, and around 0.01% radiation. The CMB is the earliest picture of the Universe that we have, and as long as we’re using light to take a picture, it is likely the earliest picture we ever can have. And even back then, just 380,000 years after the Big Bang, the evidence for dark matter is written all over it.
Ethan Siegel is the author of Beyond the Galaxy and Treknology. You can pre-order his third book, currently in development: the Encyclopaedia Cosmologica.