Why physicists now question the fate of the Universe
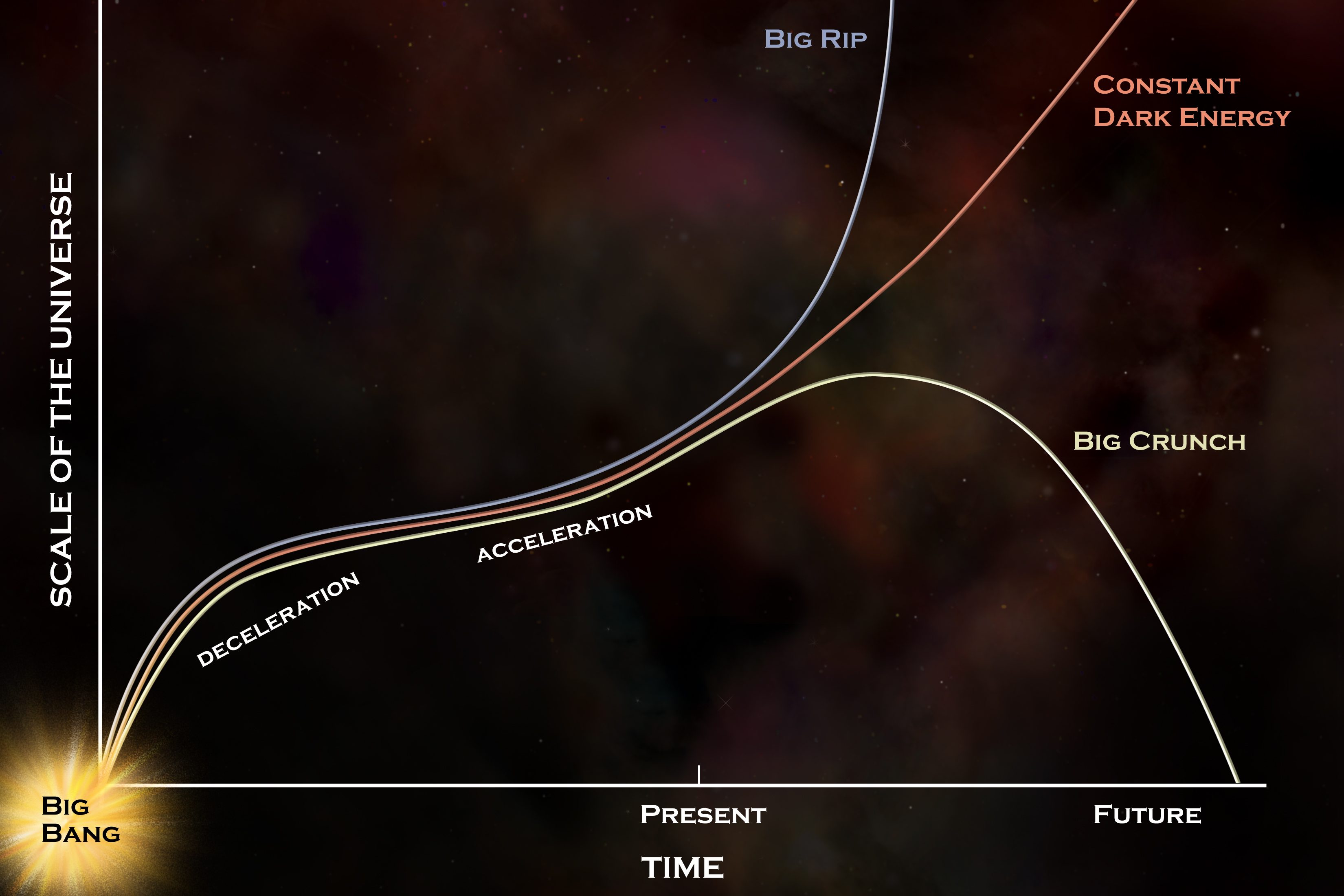
- If you can measure the Universe’s expansion rate today and how its expansion rate has changed over its history, you should be able to determine what it’s made of and, therefore, what its ultimate fate will be.
- Starting in the late 1990s, combinations of large-scale structure data, supernova data, and CMB data all pointed toward a single picture: a dark energy-dominated Universe that would end in a “big freeze.”
- Now in 2024, however, the picture has gotten a lot murkier. The Hubble tension, along with new results from DESI, throw our consensus picture into doubt. Here’s why we now question the fate of the Universe.
Ever since we first recognized that the Universe was expanding, one question has dominated the minds of those who study it: how will it all end? Today, we recognize that our Universe began from a much smaller, denser, hotter, and more uniform state: a state that we identify with the hot Big Bang. Over time, that Universe has expanded, cooled, and evolved, but also gravitated: clumping and clustering to form an intricate cosmic web of structure across millions and even billions of light-years. Today, when we use our powerful instruments to look out at the Universe — nearby, at intermediate distances, and to the greatest distances we can measure — we learn what the Universe is made of, and draw conclusions about what its ultimate fate would be.
In the 1960s, we found unambiguous evidence supporting the hot Big Bang. In the 1970s and 1980s, it became clear that our Universe also contained large amounts of dark matter: more dark matter than normal matter, in fact. And starting in the 1990s, we discovered that the Universe — and its expansion rate — were both dominated by dark energy, which behaved as a form of energy inherent to space itself. All of this led us to an astounding conclusion: that based on what we know about the laws of physics and the contents of our Universe, our fate would be to expand forever, with dark energy remaining at a constant energy density for eternity.
But now, with new state-of-the-art data, we cannot be sure. Here’s why physicists are no longer certain about the fate of the Universe.
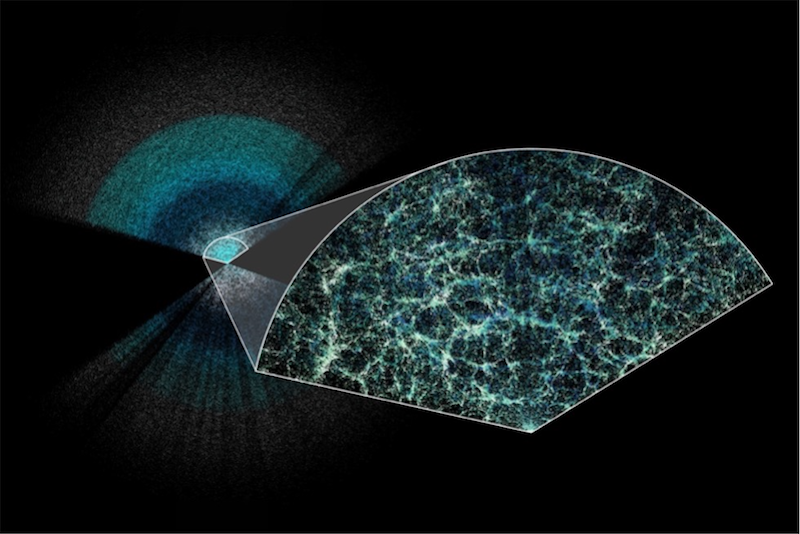
Back in the 1990s, it became pretty clear that something about our picture of the Universe wasn’t fully adding up. You see, the law of gravity that governs the Universe — Einstein’s general relativity — tells us that if we can measure:
- what the Universe is made of,
- plus how quickly the Universe is expanding today,
we can use that information to extrapolate all sorts of information about the Universe itself. We can use it to find out the age of the Universe, and the amount of time that’s elapsed since the start of the hot Big Bang. We can determine how the density of the various species of energy — normal matter, dark matter, radiation, neutrinos, spatial curvature, as well as anything else — has evolved over cosmic history. And, if we understand how these different species of energy behave as the Universe expands, we can even use this information to predict the fate of the Universe.
At the start of the 1990s, several pieces of information weren’t quite adding up. The Universe was expanding at a rate that, if all that were present within it were matter and radiation, indicated its age was young: between 9 and 12 billion years, whereas there were stars and star clusters that were closer to 14-16 billion years old. The total amount of matter in the Universe — normal matter, dark matter, and even neutrinos all combined — only added up to about ~30% of the critical density. And that little bit of information held tremendous implications for the fate of our Universe.

You see, in the science of physical cosmology, there are two competing factors that are at play in the expanding Universe. On one hand, there’s the initial expansion rate: the rate at which space itself is expanding at the start of the hot Big Bang, when the Universe is at its hottest, densest, and most uniform. But on the other hand, there are all of the effects of gravity: the tendency for matter and energy to attract, curve, and distort spacetime, and to try to “pull” the Universe back together in some sense. If your Universe consists only of matter-and-radiation and starts off by expanding, then there are three possibilities as to the eventual fate of the Universe.
- The expansion rate is too great for the matter and radiation in the Universe to overcome, and even though gravity can slow down the cosmic expansion, the Universe continues to expand forever, leading to a heat death scenario, or what’s known as a Big Freeze.
- The opposite can occur: where there’s enough matter and radiation to out-gravitate the initial expansion. The Universe expands but gravity slows it down, eventually brings it to a halt, and then reverses the expansion into contraction. This scenario ends in a reverse of the Big Bang: a Big Crunch.
- Or, you can be in perfect balance between the two scenarios: the “Goldilocks” case. One more atom in the Universe would lead to cosmic recollapse, but in the absence of that atom, the Universe just keeps coasting as the expansion rate drops asymptotically to 0. This is the case known as a critical Universe.

But toward the latter part of the 1990s, we got a few critical pieces of information about our Universe that came as a surprise to many. First, a heroic set of efforts among those who study distant supernovae led to a revolutionary set of measurements: measurements that plotted out the redshift-versus-distance relation of standard objects — type Ia supernovae — whose intrinsic brightness could be known just by measuring the light from these supernovae. The amount that the light redshifts by tells us how much the Universe has expanded since that light was emitted, while a greater distance to the object allows us to look farther back in time. With these pieces of evidence together, we can understand how the Universe has expanded, and therefore, we can learn what makes it up.
Right around the same time, we started getting measurements of imperfections in the leftover glow from the Big Bang: the cosmic microwave background, or CMB. Those measurements are particularly good at telling us what the overall curvature of space is. If the Universe were made of matter-and-radiation alone, then:
- a positive curvature would correspond to a Big Crunch,
- a negative curvature would correspond to a Big Freeze,
- and a curvature of 0 would correspond to a critical Universe.
These combined measurements — of supernova data and of the CMB — taught us something we never would have anticipated in their absence: the Universe has a curvature of 0, but not enough matter-and-radiation to bring about the expected “critical Universe” scenario. Instead, there was another ingredient in play: one that would increase the age of the Universe from 9-to-12 billion years to more like 14 billion years (13.8 billion, to be precise).
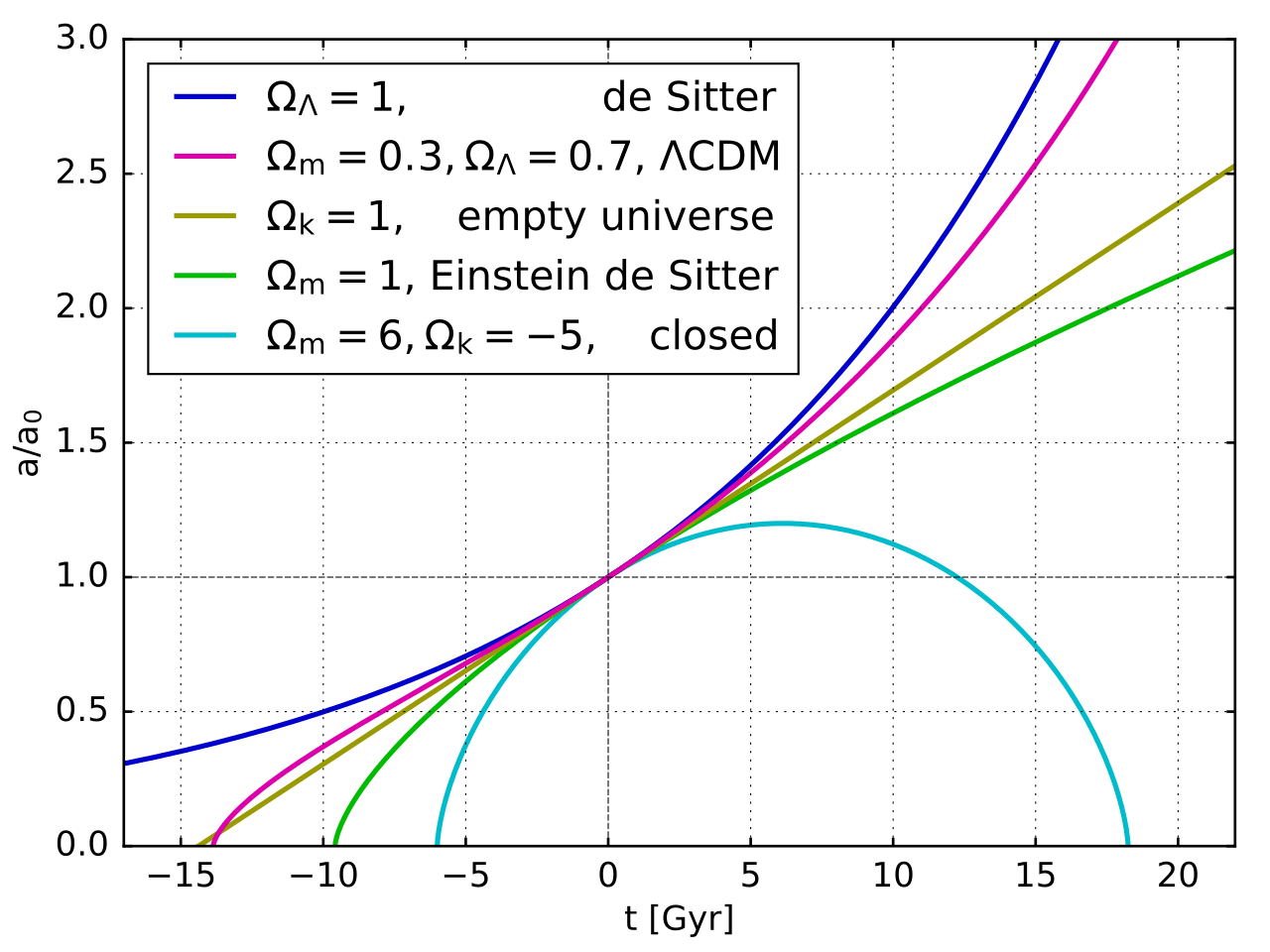
That realization, driven by measurements, was what led to the widespread acceptance of dark energy: a new species of energy in our Universe. Something else had to be there besides matter-and-radiation, otherwise the Universe couldn’t be spatially flat. That something else couldn’t be like radiation, nor could it be like matter, nor even like spatial curvature, but rather had to be a different species entirely: one that didn’t oppose the ongoing expansion, but instead contributed to it. The supernova data, after all, was indicating that a distant object, if you were to continue observing it over long periods of time, would now appear to recede from us at faster and faster rates, with its redshift increasing over time.
There had been exotic forms of energy considered before that could do this. If the Universe were dominated by cosmic strings, for instance, a distant object would recede at a constant rate. If the Universe were dominated by domain walls, a distant object would recede at an increasing rate over time: corresponding to an accelerating Universe. And if the Universe were dominated by a form of energy that was inherent to space itself — like Einstein’s cosmological constant or the vacuum energy from quantum field theories — a distant object would recede faster and faster over time: even more severely than in the domain walls case.

This led to a shift in the longstanding cosmic quest: instead of determining the fate of the Universe, we now had to determine what this new form of energy — dubbed dark energy — actually was. In general relativity, there’s a relationship between any species of energy’s energy density, ρ, and its pressure, p. For matter, it’s considered pressureless, where p = 0. For radiation, the pressure is positive and equal to a third of the energy density: p = +⅓ρ. In order to cause a distant object to recede faster and faster over time, the pressure must be more negative than the case of cosmic strings, where p = -⅓ρ. That number that related pressure to energy density for any species is typically called w, where p = wρ, and that meant the goal for dark energy became determining what its w actually is. (For those wondering, w for domain walls is -⅔ and w for a cosmological constant is -1.)
Over the 2000s and early 2010s, the supernova data strengthened tremendously, indicating that w is very close to -1. During that same time, scientists began to robustly measure a feature imprinted from the oscillation of normal matter, dark matter, and radiation in the early Universe: a “bump” in galaxy correlations known as baryon acoustic oscillations, or BAO. BAO measurements, by looking at how galaxies cluster in the Universe, allow us to measure how the Universe expands over time as well, and can give us an independent measurement of w as well. From BAO and supernova data combined, it looked like w = -1.00, with an uncertainty of only ± 0.04 or so.

If dark energy truly has w = -1, exactly, then it behaves as a cosmological constant, and the fate of the Universe is known: it will undergo a Big Freeze, and the various objects that were not yet gravitationally bound when dark energy became the dominant factor in cosmic expansion (around 6 billion years ago) will eternally recede from one another. The Universe will become cold and empty, and eventually bound structures like galaxies, galaxy groups, and even galaxy clusters, would not only become isolated, but would largely decay away due to internal gravitational ejections over time. Our Universe would become cold and lonely.
All of that is only true under a certain set of assumptions, however; we are assuming that:
- the dark energy equation of state is exactly w = -1,
- and that dark energy doesn’t vary in strength either across space or over time.
In the absence of any compelling data suggesting that dark energy isn’t a pure cosmological constant, this is a reasonable assumption to make. But there are two pieces of evidence that very much suggest otherwise. The first came about in the late 2010s and has been strengthened throughout the 2020s: the observation that the rate at which the Universe is expanding today — what we conventionally call the Hubble constant — depends on whether you use an “early relic” method to measure it, like the CMB or BAO features, or whether you use a “distance ladder” method, such as parallax, Cepheids, and supernovae to measure it.
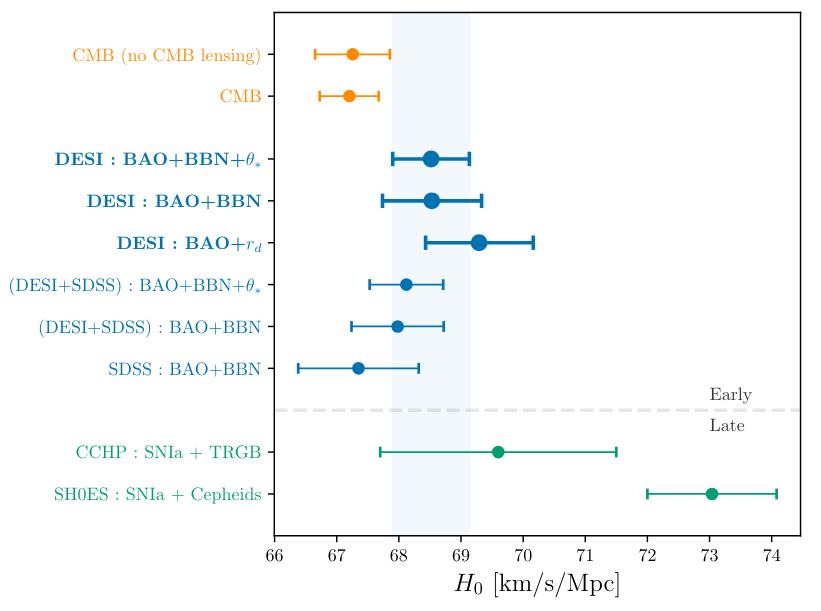
The first class of methods, all relying on some sort of early relic, all give answers that are somewhere around 67 km/s/Mpc, with an uncertainty of just ± 1 km/s/Mpc to them. The second class of methods, relying on objects that emitted light at late times and that light then arrived at our eyes, give answers that are closer to 73 km/s/Mpc, again with an uncertainty of just ± 1 km/s/Mpc to them. The results obtained are all consistent between groups using an “early relic” or “distance ladder” methodology, but inconsistent between the two groups. It has led many to consider exotic cosmologies that can contain exotic or even evolving species of energy, as those are potential candidate solutions for resolving this so-called Hubble tension.
However, an even more recent observation has given us further reason to reconsider the fate of the Universe: new data, containing spectroscopic information about more than six million galaxies, has just been released by the Dark Energy Spectroscopic Instrument (DESI) collaboration, and it gives us our strongest indication yet that dark energy may be evolving over time. Their value for the Hubble constant agrees with other “early relic” methods; they find a Universe made of only ~30% matter (and about ~70% dark energy), but when they add in the CMB and/or supernova data sets, they then find that dark energy doesn’t appear to have a constant energy density over time, after all.
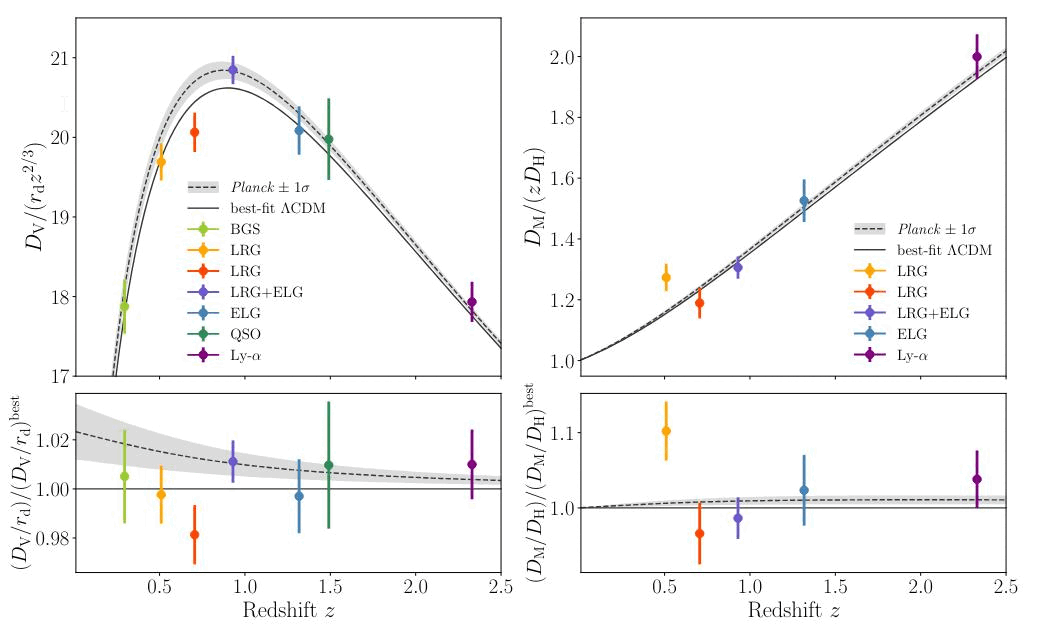
In fact, what their data is most consistent with is the following scenario: that dark energy began as though it were a cosmological constant, with w = -1 and not changing, and then, about 7 billion years ago, slowly and slightly began weakening. It may even support or favor scenarios in which w is slightly “less negative” than -1, such as w ~ -0.8, and where it is evolving by becoming even less negative as time goes on. In particular, it’s not the ultra-distant galaxies that are driving the departure from the expected “vanilla” version of dark energy, where it’s just a plain old cosmological constant, but the galaxies found relatively nearby: whose light is arriving after a journey of between 4 billion and 8 billion years of traveling through space.
It’s as though this baryon acoustic oscillation (BAO) feature, easily detectable in large-area surveys of the sky that spectroscopically measure the properties of galaxies, hasn’t been lengthening — particularly in recent times — as much as one would expect it to in a Universe where dark energy is a pure cosmological constant. Although the DESI data set is poised to triple in the relatively near future, and observatories such as ESA’s Euclid, NASA’s Nancy Roman, and the NSF’s Vera Rubin will serve as valuable independent cross-checks of the DESI team’s work, we now are compelled to seriously consider that dark energy may not be as simple and straightforward as we once anticipated at the dawn of the 21st century.
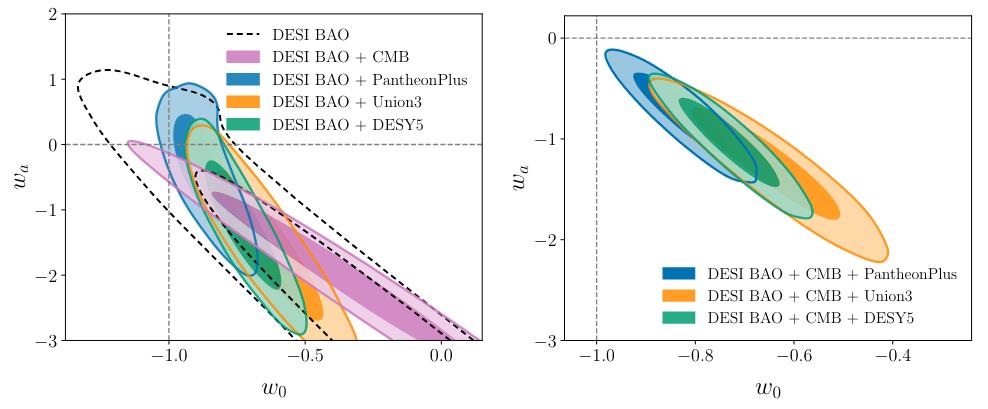
Contrary to the way almost everything else in the world works, scientists would be overjoyed to be shown that our simple picture for how dark energy worked — that it was just a cosmological constant inherent to space that never changed over time — was wrong, and failed to match the reality of what we were observing. What we call the Standard Model of cosmology, or the ΛCDM model, is almost certainly only an approximation to our true physical reality, albeit an approximation that’s served us very well so far. If we can find an observational test where that approximation is no longer a good one, that’s almost certainly a clue that will lead us to a richer understanding of our Universe as it actually is.
Perhaps dark energy isn’t truly a constant, after all. Perhaps it is something that changes and evolves with time. If so, our cosmic fate could dramatically differ from what we typically suppose. If dark energy strengthens and becomes more negative with time, it could lead to a Big Rip. If it weakens and becomes more positive, it could potentially stop the Universe from accelerating and may even revive the possibility that we’ll recollapse and end in a Big Crunch. With years of new DESI data just waiting to be analyzed, and tens of millions of objects to be added to their upcoming catalogs, we might see what comes of these hints sooner rather than later. In the meantime, it’s of paramount importance that we keep our minds open to all of the possibilities that the data still admits. After all, the Universe may turn out to be a stranger place than anyone has imagined so far.