Ignition achieved! Nuclear fusion power now within reach

- For the first time in the history of nuclear fusion, ignition has been achieved: where the energy released from fusion reactions exceeds the energy inputted to trigger them.
- Achieving ignition, or passing the breakeven point, is one of the key goals of nuclear fusion research, with the eventual goal of achieving commercial-scale nuclear fusion power.
- However, achieving this goal is only one more step toward the true dream: of powering the world with clean, sustainable energy. Here’s what we all should know.
For decades, the “next big thing” in terms of energy has always been nuclear fusion. In terms of sheer potential for power generation, no other energy source is as clean, low-carbon, low-risk, low-waste, sustainable, and controllable as nuclear fusion. Unlike oil, coal, natural gas, or other fossil fuel sources, nuclear fusion won’t produce any greenhouse gases like carbon dioxide as waste. Unlike solar, wind, or hydroelectric power, it’s not reliant on the availability of the needed natural resource. And unlike nuclear fission, there’s no risk of a meltdown and no long-term radioactive waste produced.
Compared to all other alternatives, nuclear fusion is clearly the optimal solution for generating power on Earth. The biggest problem, however, has always been this: even though nuclear fusion reactions have been achieved by a variety of means, there’s never been a sustained fusion reaction that’s achieved what’s known as either:
- ignition,
- net energy gain,
- or the breakeven point,
where more energy is produced in a fusion reaction than was used to ignite it. For the first time in history, that milestone has now been achieved. The National Ignition Facility (NIF) has reached ignition, a tremendous step toward commercial nuclear fusion. But that doesn’t mean we’ve solved our energy needs; far from it. Here’s the truth of how it’s truly a remarkable achievement, but there’s still a long way to go.

The science of nuclear fusion is relatively straightforward: you subject light atomic nuclei to the conditions of high temperature and high density, triggering nuclear fusion reactions that fuse those light nuclei into heavier ones, which releases energy that you can then harness for the purposes of generating electricity. Historically, this has been achievable primarily via one of two means:
- either you create a magnetically confined, low-density plasma that enables these fusion reactions to occur over time,
- or you create an inertially confined, high-density plasma that triggers these fusion reactions in one tremendous burst.
There are hybrid methods that use a combination of both, but these are the two major ones being researched by reputable institutions. The first method has been leveraged by Tokamak-type reactors such as ITER to achieve nuclear fusion, while the second method has been leveraged by omnidirectional laser shots to trigger fusion from tiny, light-element-rich pellets, such as the National Ignition Facility (NIF). Over the past thirty years or so, the records for “whose been closest to breakeven” has gone back and forth between these two methods, but in 2021, inertial confinement fusion at the NIF surged ahead, achieving near-breakeven energy outputs by some metrics.

Now, a further improvement has brought inertial confinement fusion truly ahead of its primary competitor: liberating 3.15 megajoules of energy from only 2.05 megajoules of laser energy delivered to the target. Since 3.15 is greater than 2.05, this means that ignition, breakeven, or net energy gain — dependent on your favored term — has at last been achieved. It’s a huge milestone that was enabled, of all things, by the research behind 2018’s Nobel Prize in Physics, which was awarded for advances in laser physics.
The way lasers work is that specific quantum transitions that occur between two distinct electron energy levels in matter are repeatedly stimulated, resulting in the emission of light of precisely the same frequency, over and over again. You can increase the intensity of your laser by better collimating the beam and by using a better amplifier, which allows you to create a more energetic, powerful laser.
But you can also make a more intense laser by not emitting your laser light continuously, but by controlling the power and pulse frequency of your laser. Rather than continuous emission, you can “save up” that laser light and emit all of that energy in one single, short burst: either all-at-once or in a series of high-frequency pulses.

Two of 2018’s Nobel Laureates — Gérard Mourou and Donna Strickland — solved exactly this problem with their Nobel-winning research. In 1985, they published an article where they not only detailed how to create an ultra-short, high-intensity laser pulse in a repetitive fashion, but they were able to do it without harming or overloading the amplifying material. The four-step process was as follows:
- First, they created these relatively standard laser pulses.
- Then, they stretched the pulses in time, which reduces their peak power and makes them less destructive.
- Next, they amplified the time-stretched, reduced-power pulses, which the material used for amplification could now survive.
- And finally, they compressed the now-amplified pulses in time.
The shortening of the pulse, in time, means that more light of greater intensity became packed together in the same space, leading to a massive increase in the intensity of the pulse. This technique, known as Chirped Pulse Amplification, is now used in a wide variety of applications, including millions of corrective eye surgeries performed each year. But it also has another application: to the lasers used to create the conditions needed to achieve inertial confinement fusion.

The way inertial confinement fusion works at the NIF is truly an example of the success of the “brute force” approach to nuclear fusion. By taking a pellet of fusible material — typically a mix of light isotopes of hydrogen (like deuterium and tritium) and/or helium (like helium-3) — and shooting them with high-powered lasers from all directions at once, the temperature and density of the nuclei inside the pellet increases tremendously.
In practice, this record-breaking shot at NIF leveraged 192 independent, high-powered lasers firing all at once on the target pellet. The pulses arrive within fractions-of-a-millionth-of-a-second of one another, where they heat the pellet to temperatures of over 100 million degrees: comparable to densities and exceeding energies found in the center of the Sun. As the energy propagates from the outer part of the pellet toward its core, fusion reactions are triggered, creating heavier elements (like helium-4) from lighter elements (such as deuterium and tritium, i.e., hydrogen-2 and hydrogen-3), releasing energy in the process.
Even though the timescale for the entire reaction can be measured in nanoseconds, the blast from the lasers plus the surrounding mass of the pellet is sufficient to briefly confine (via inertia) the plasma to the pellet’s core, enabling large numbers of atomic nuclei to fuse during this time.

There are a few reasons why this latest step really is an exciting — even a game-changing — development in the quest for nuclear fusion power. Since the 1950s, we’ve known how to trigger nuclear fusion reactions and to generate more energy than we inputted: through a thermonuclear detonation. That type of reaction, however, is uncontrolled: it can’t be used to create small amounts of energy that can be harnessed to produce usable power. It simply goes off all at once, resulting in an enormous and highly volatile release of energy.
However, the results of those early nuclear tests — including underground tests — that we could easily produce breakeven (or greater-than-breakeven) energy outputs if we were capable of injecting 5 megajoules of laser energy equally around a pellet of fusible material. At the NIF, earlier attempts at inertial confinement fusion only had 1.6 megajoules and, later, 1.8 megajoules of laser energy incident on the target. These attempts fell well short of the breakeven point: by factors of hundreds or more. Many of the “shots” failed to produce fusion entirely, as even slight imperfections in the sphericity of the pellet or the timing of the laser strikes rendered the attempt a failure.
As a result of the disconnect between NIF’s capabilities and the demonstrated energy needed for true ignition, researchers at the NIF lobbied congress over the years for additional funding, with the hopes of building what they knew would work: a system that reached 5 megajoules of incident energy. But the level of funding that would be required for such an endeavor was deemed prohibitive, and so the NIF scientists had to get very clever.
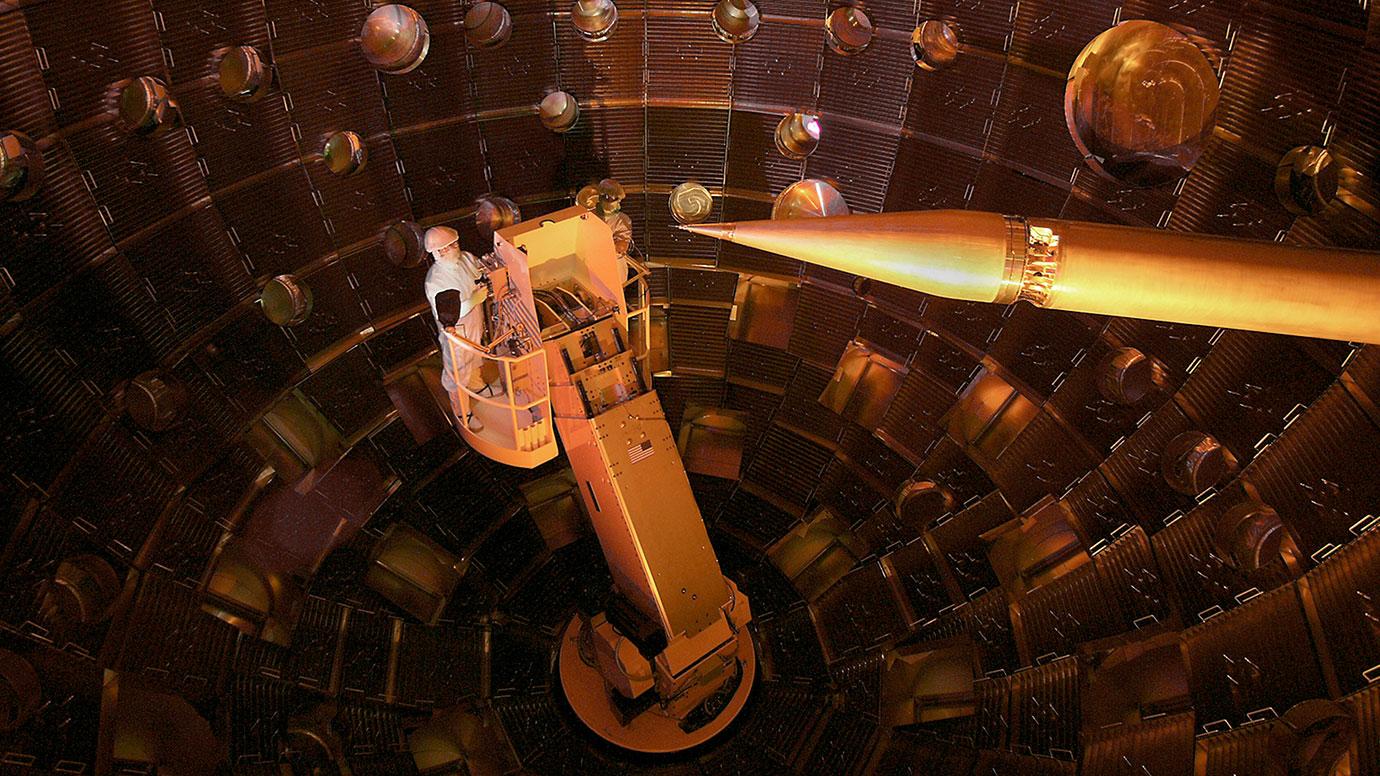
One of the main tools they relied on were detailed simulations for how the fusion reactions would progress. Early on, and even in recent years, there have been many vocal members of the fusion community that worried these simulations were unreliable, and that performing underground nuclear tests was the only robust way to collect the needed physical data. But these underground tests create radioactive fallout (that usually, but not always, remains confined to the underground cavity), as you might expect whenever nuclear reactions occur in the presence of already-heavy elements. Producing long-lived radioactive material is never desired, and that’s not only a drawback of underground nuclear tests, but of the magnetic confinement fusion approach as well.
But inertial confinement fusion, at least when performed on a pellet of hydrogen-based fuel for short periods of time, doesn’t have that problem at all. No long-lived, heavy radioactive elements are produced: something that simulations and real-world tests both agree on. Simulations had indicated that perhaps, with as little as 2 megajoules of laser energy incident on a target with the right parameters, a greater-than-breakeven fusion reaction could be achieved. Many were skeptical of this possibility, and of the simulations in general. After all, when it comes to any physical process, only data collected from phenomena in the real world can guide the way.
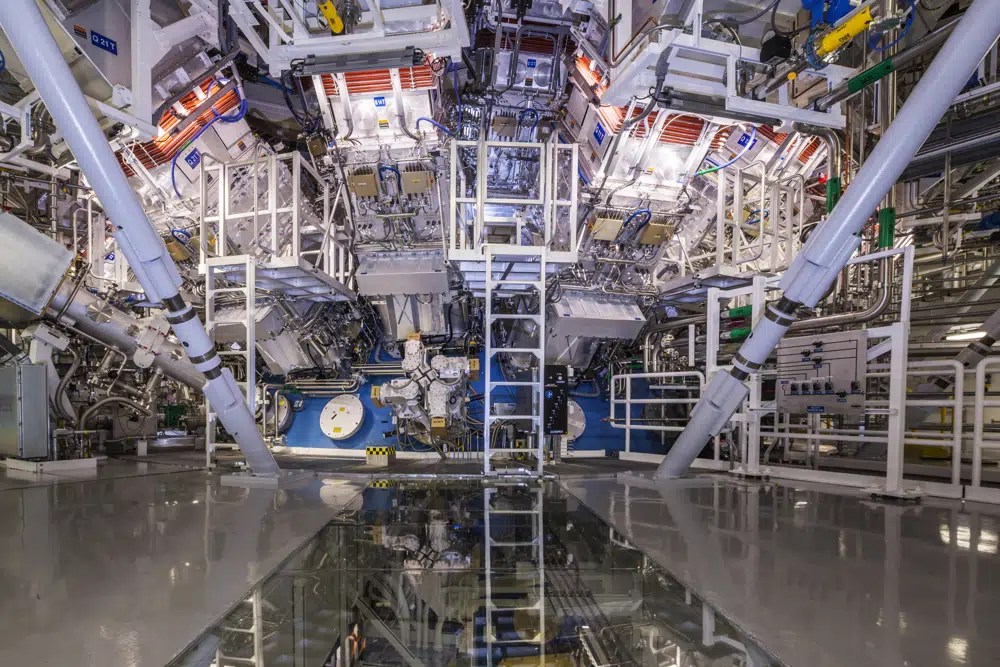
That’s why this recent NIF achievement is really, truly something to marvel at. There’s a saying among scientists who work on nuclear fusion: that energy washes away all sins. At 5 megajoules of laser energy incident on the pellet, a large fusion reaction would be guaranteed. At 2 megajoules, however, everything needed to be precise and pristine.
- The optical lenses, which focused the lasers, needed to be completely impurity and dust-free.
- The pulses from the nearly 200 lasers needed to arrive simultaneously, within less than a millionth of a second, at the target.
- The target needed to be perfectly spherical, with no discernible imperfections.
And so on. Just about two years ago, a remarkable laser “shot” was conducted at the NIF, with the laser energy raised to 2 megajoules for the first time. It produced some ~1.8 megajoules of energy (nearly reaching the breakeven point) with all of these conditions met, a strong piece of evidence in support of what the simulations were predicting. But this latest achievement, where the energy was raised by just a tiny bit (to 2.1 megajoules), produced a much increased 3.15 megajoules of energy, even though they used a less perfectly spherical and thicker target for their pellet. They were able to confirm the predictions and the robustness of their simulations, while simultaneously demonstrating the truth behind the notion that energy really does wash away the sins of imperfections.
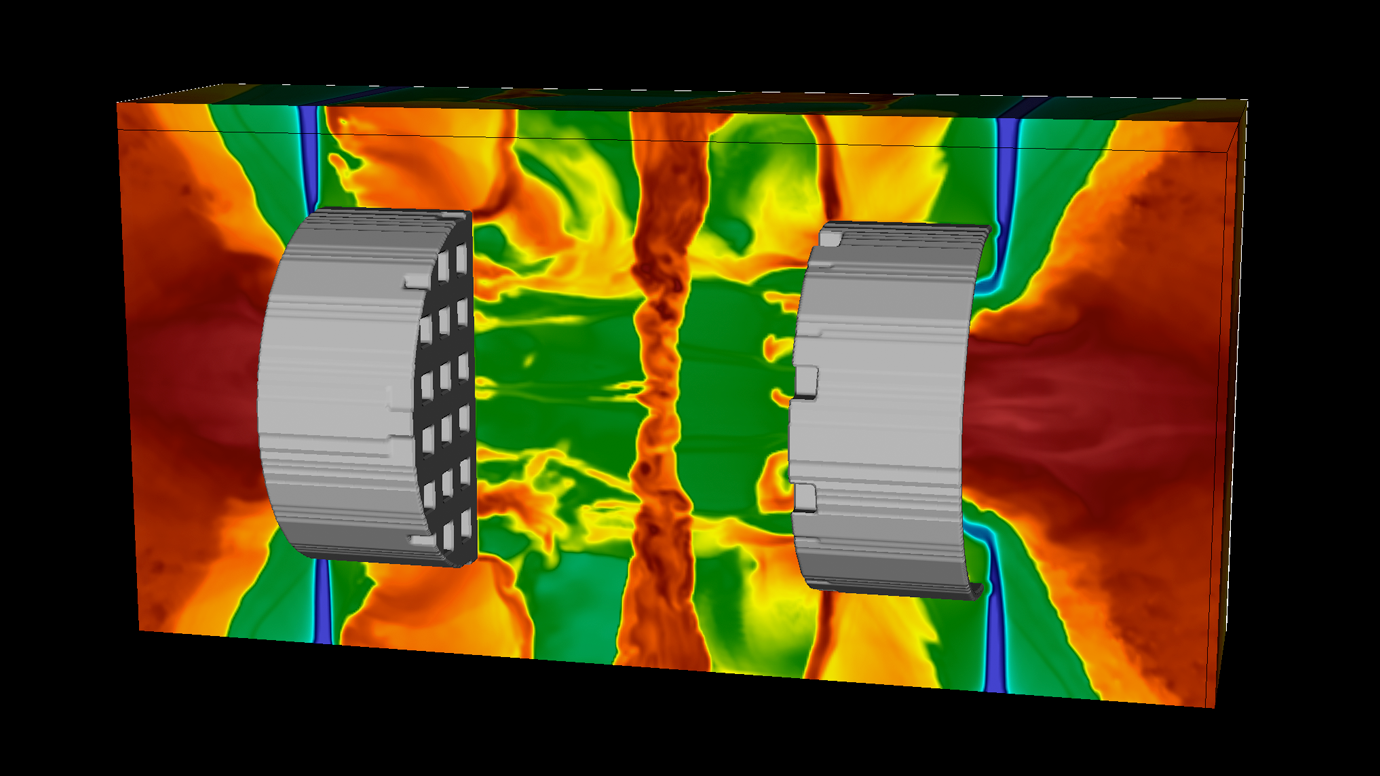
Nuclear fusion has been studied very seriously with a view toward commercial-scale power production for over 60 years, but it’s this experiment that marks the very first time in history that the vaunted breakeven point has been passed.
However, that doesn’t mean the climate/energy crisis is now solved. Quite to the contrary, although this is certainly a step worth celebrating, it’s just another incremental improvement toward the ultimate goal. To be clear, here are the steps that must all be achieved in order for commercial-scale fusion power to become viable.
- Nuclear fusion reactions must be achieved.
- More energy must arise from those reactions than was inputted to trigger those reactions.
- The energy that arises must then be extracted, and transformed into a form of energy that can then be either stored or transmitted: in other words, put to good use.
- The energy must be produced either steadily or repeatably, so that it can provide power-on-demand, the way we’d demand it for any other type of power plant.
- And the materials and equipment consumed and used/damaged during the reaction must be replaced and/or repaired on timescales that don’t hinder the recurrence of that reaction.
After being stuck on step 1 for over half-a-century, this recent breakthrough finally gets us to step 2: the achievement of what we call “ignition.” For the first time, the next steps are not subject to scientific doubt; they’re simply a matter of the engineering details needed to bring this now-proven technology to life.
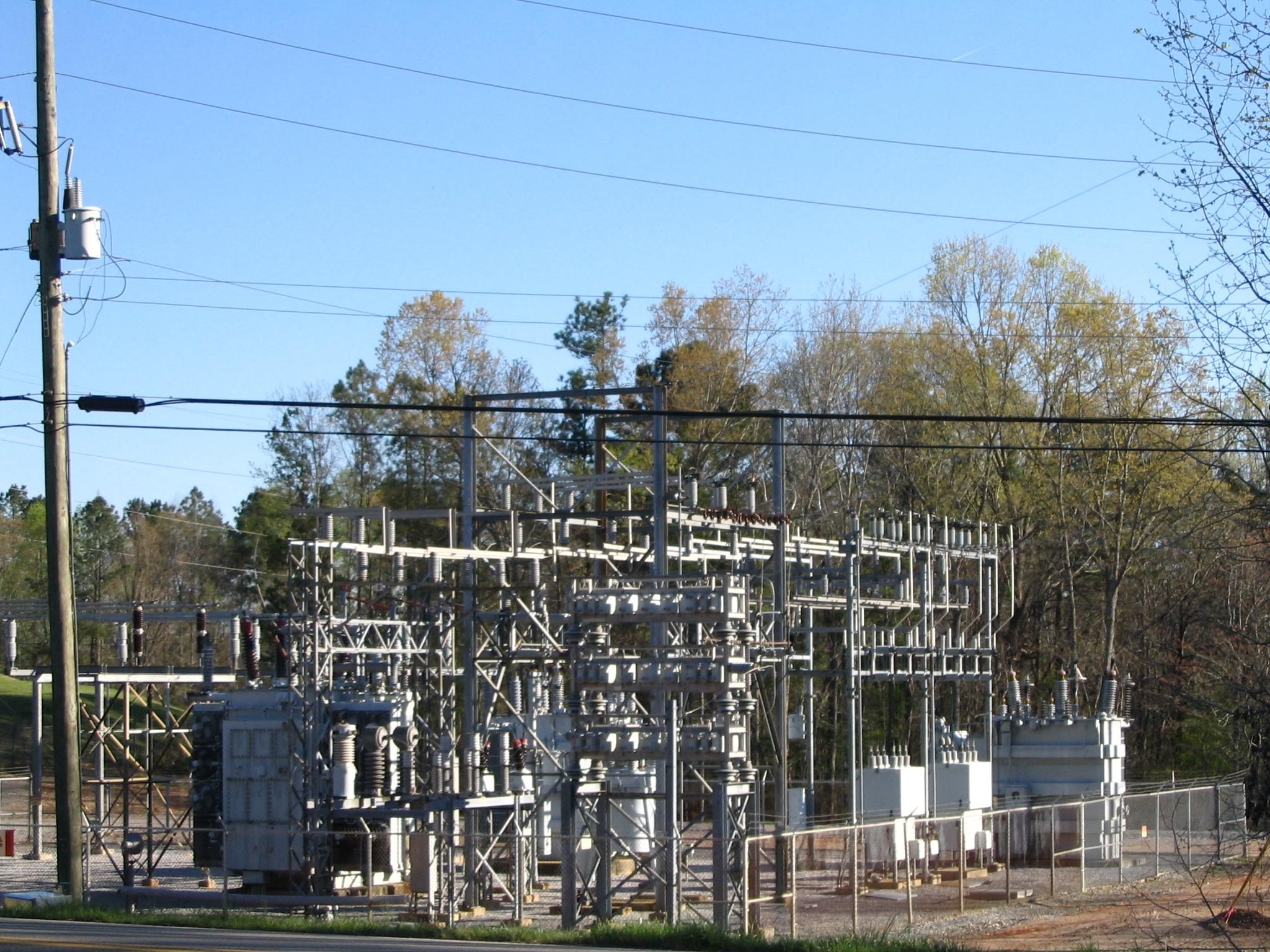
If you’ve thought about fusion power, chances are you’ve encountered the old adage, “Viable fusion power is 50 years away… and always will be.” But according to Professor Don Lamb at the University of Chicago, that’s definitely no longer the case. When I asked him about this issue, he stated:
“That was then and this is now. As long as there were physical processes that we didn’t understand until we did it robustly, no one could be sure that we’d be able to [achieve ignition]. The physics of plasmas is incredibly rich, as is [the physics of] lasers.
Nature fought back hard; as soon as you dealt with one physical process, nature said, ‘A ha! Here’s another!’ Because we didn’t understand all the physical processes that stood in our way, we’d think, ‘Oh, I handled this problem, so it’ll be 50 years from now,’ and it just kept going like that ad infinitum. But now we can say, ‘Oh, nature, you’re out of tricks, I’ve got you now.'”
In other words, before we achieved ignition — i.e., before we passed the breakeven point — we knew there were going to be fundamental science issues we had yet to uncover. But now those issues have been identified, dealt with, and are behind us. There are still plenty of developmental issues to face and overcome, but from a scientific perspective, the problem of passing the breakeven point and generating more energy than we put in has been overcome at long last.

There are a myriad of takeaway points from this new development, but here’s what I think everyone should remember about nuclear fusion as we move forward into the future.
- We really have passed the breakeven point: where the energy incident on a target — the key energy that triggers a fusion reaction — is less than the energy we get out of the reaction itself.
- That threshold is just over 2.0 megajoules of incident laser energy, far less than many who asserted 3.5, 4, or even 5 megajoules would be required to achieve the breakeven point.
- A new facility, one with lenses and apparatuses designed to withstand these new energies, must be constructed.
- A prototype energy-generation plant will need to leverage still-developing technologies: safely chargeable capacitor banks, large systems of lenses so that successive fusion-generating shots can be fired with a new set of lenses while the recently used set can be “healed,” the ability to harness and convert the released energy into electrical energy, energy storage systems that can hold and distribute the energy over time, including during the time between successive shots, etc.
- And the dream of a home fusion plant that lives in your backyard will have to be relegated to the far future; residential homes cannot handle megajoules of energy being pulsed through them, and the needed capacitor banks would create a substantial fire/explosion hazard. It won’t be in your backyard or anyone’s backyard; these fusion generating endeavors belong in a dedicated, carefully monitored facility.
Overall, now is the perfect time for a substantial investment in all of these technologies, with this achievement giving us every reason to believe that we can completely decarbonize the energy sector worldwide during the 21st century. It’s a tremendous time to be a human on planet Earth; it’s now up to us to make our investments count.
Ethan Siegel thanks Professor Don Lamb for an invaluable conversation concerning the latest NIF research.