New LHC results refute Fermilab’s “hole” in the Standard Model
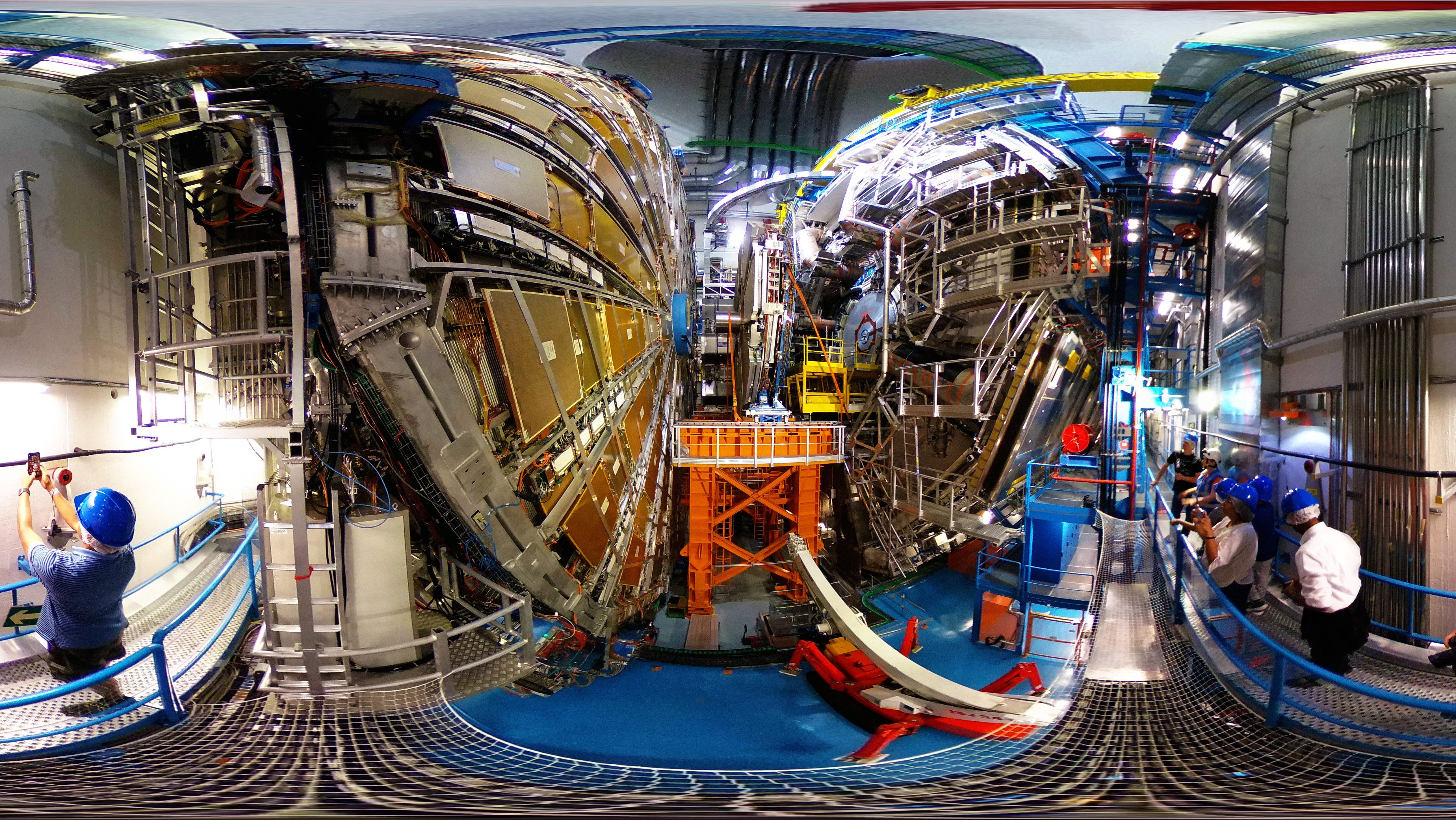
- Almost as soon as the LHC turned on and began collecting data, evidence for the last undiscovered particle of the Standard Model, the Higgs boson, began to appear.
- In the 12+ years since its discovery was announced, no new fundamental particles have appeared or been discovered, leaving us stuck with unsolved mysteries and the Standard Model that doesn’t explain them.
- In 2022, Fermilab’s CDF collaboration released a shocking claim that the W-boson mass was much higher than previously thought, which challenged everything. With new results from the LHC, the picture finally clears up.
In all of scientific history, no single theory has been tested more robustly — and still stands without a single failure — than the Standard Model of elementary particles and forces. Successfully describing every known species of quantum particle in existence as well as their properties and interactions, the Standard Model was assembled way back in the 1960s as a way of piecing together the fermions (quarks and leptons) that make up matter and antimatter into a framework that details their interactions through the exchange of bosons (the photon, W-and-Z bosons, gluons) that mediate the electromagnetic, weak, and strong nuclear forces, as well as the mechanism that gives all particles their rest masses (leading to the Higgs boson as well).
The interesting thing about the Standard Model is this: it’s a constrained theory. If you understand how the various forces and interactions work, and you measure the masses of every species of particle in the Standard Model, you’ll find that either:
- they are all in mutual agreement in a fashion consistent with the Standard Model,
- or they present a tension with the Standard Model, indicating that something novel — beyond the Standard Model — must be contributing as well.
In 2022, researchers from the CDF collaboration at Fermilab, the world’s most powerful particle accelerator before the Large Hadron Collider (LHC) at CERN, shocked the world by presenting evidence for a W-boson mass that fell into the second category: disagreeing with the Standard Model’s predictions. But the LHC gets to weigh in on this, too. Now, in 2024, the results are in, and it looks like the Standard Model survives, after all. Here’s a window into the current state of affairs.
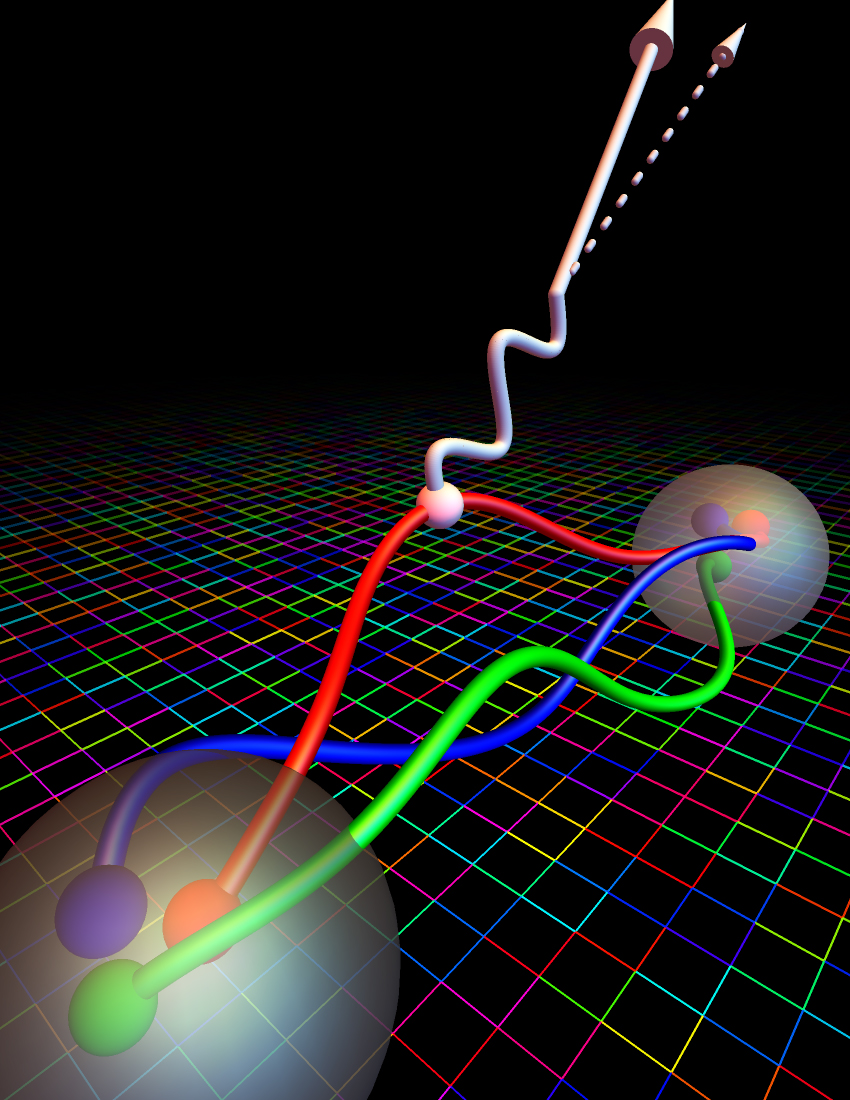
W-bosons and the CDF surprise
The particle known as the W-boson, which comes in both positively and negatively charged varieties, had long been suspected to be a heavy, massive, short-lived fundamental particle responsible for changing the species/identity of quarks and leptons: i.e., responsible for the weak decays. Although theorized way back in the 1960s, its massive nature required a large set of advancements in accelerator and detector technologies before it would finally yield to direct detection in 1983: when the UA1 and UA2 collaborations, led by Carlo Rubbia and Pierre Darriulat respectively, announced its existence at CERN. The mass of the W-boson is highly dependent on the Higgs mechanism, as it’s the broken electroweak symmetry that gives the W-boson its mass.
Because the masses of the Standard Model particles are all related, the expected mass of the W-boson depends on the masses of the other particles, with arguably the most important constraints coming from the mass of the top quark, itself only discovered in 1995 at Fermilab. Initially, early evidence indicated the top quark’s mass (in terms of its energy equivalent) could be anywhere from about 165 GeV up to 180 GeV, which would constrain the W-boson to be no lower than 80.33 GeV (for a low-mass top quark) and no greater than 80.40 GeV (for a high-mass top quark).
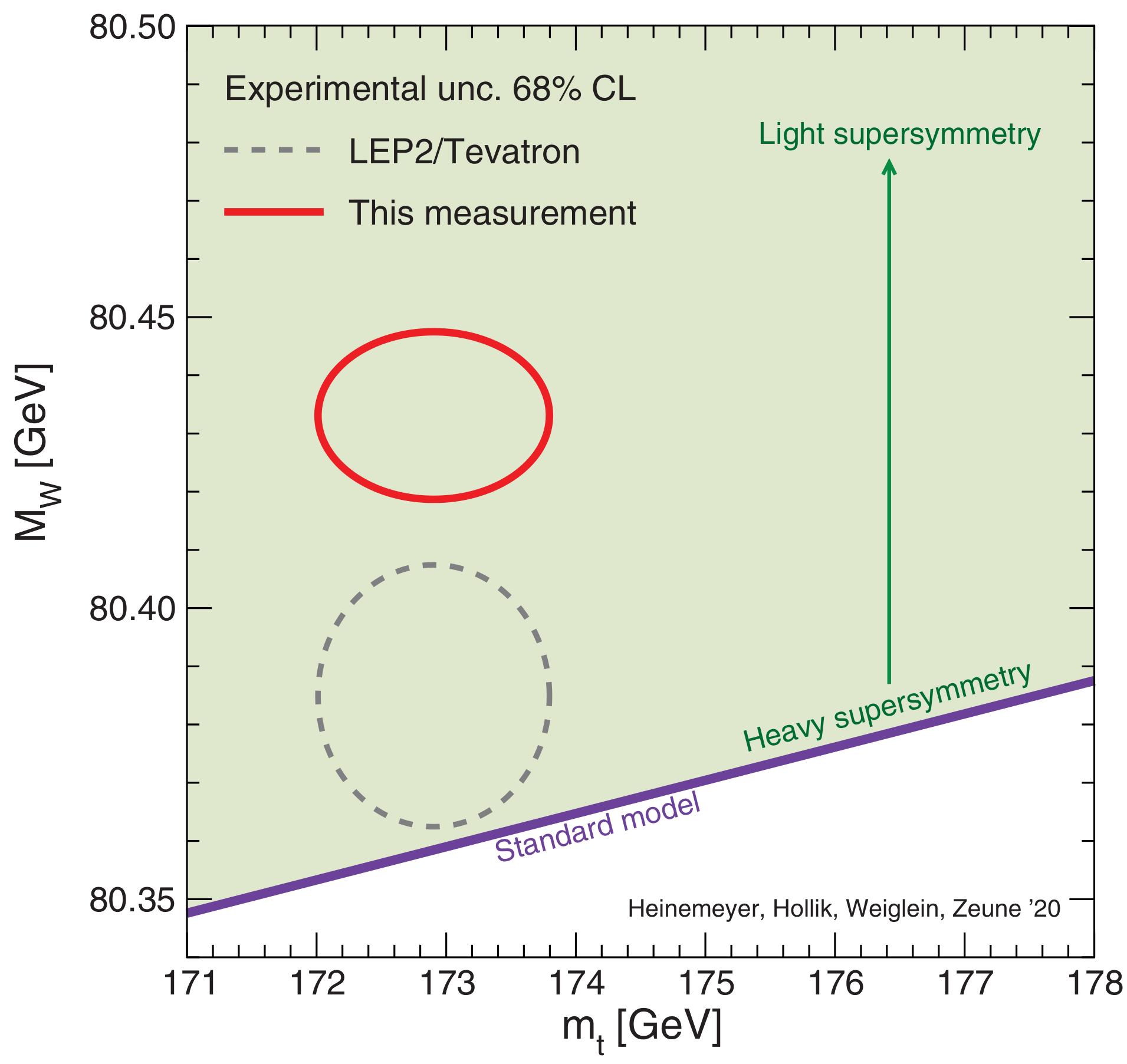
But what the CDF collaboration experimentally measured, with the smallest uncertainties of all-time, was a mass of 80.433 GeV, with an uncertainty of just 0.0094 GeV attached to it. If the top quark mass were truly in the range that all experiments had previously indicated (between 170-175 GeV) and the Higgs boson were truly where the evidence placed it (at around 125 GeV), then this posed an enormous puzzle for the Standard Model. There isn’t a whole lot of “wiggle-room” for these masses to be different than the Standard Model’s predictions, as so many alternative, beyond-the-Standard-Model scenarios had already been ruled out by existing data. Either:
- the CDF measurement was wrong,
- several other collaborations made errors or missed a key piece of evidence,
- or this CDF result was an indication that something was wrong with the Standard Model.
No one has ever found any errors with the CDF analysis, although it will be several years before the LHC can match and check the CDF collaboration’s precision. No errors or overlooked evidence has been discovered elsewhere either, leading some to suspect — or at least to hope — that perhaps this was a hint that new, beyond-the-Standard-Model physics would be within range of present-day colliders.

The rest of the world: before CDF
It wasn’t like the CDF results, released in 2022, were the first “good” measurements of the W-boson’s mass. Since its discovery in 1983, various collider experiments — using electron-positron collisions, proton-antiproton collisions, and proton-proton collisions — had all managed to create large numbers of W-bosons, measure their decay products, and use sophisticated detectors to reconstruct properties of the W-boson near the collision point. Those properties included the W-boson’s mass. In early 2022, prior to the release of the CDF results, the world average was that the W-boson’s mass was 80.379 GeV, with an uncertainty of just 0.012 GeV.
That was at least close to the Standard Model’s predicted mass of 80.36 GeV, although it favored a slightly higher mass for the top quark (178 GeV) compared to what had been measured up to that time (173 GeV). However, if the CDF results were correct, with the W-boson having a mass of 80.433 GeV, then there was no reasonable way to reconcile this mass with the Standard Model and the rest of the existing data. If the CDF results were correct, this suggested something remarkable, such as:
- supersymmetry exists and the SUSY particles are light enough to appear at the LHC,
- the Higgs boson is not actually a fundamental scalar but is actually a composite particle,
- or there’s at least one new particle out there that couples to the Standard Model particles and has not been directly detected.
But before we start considering exotic scenarios such as this, we have to check that the CDF results are actually correct, and indicate that something is wrong with the “standard” version of the Standard Model.

There are three major things to check:
- the mass of the top quark,
- the mass of the Higgs boson,
- and the mass of the W-boson,
as all three of these are interrelated. There are plenty of different ways to try to reconstruct the masses of these particles, depending on which detector and which decay channels are being probed by the experimental collaboration taking the data, which is why we typically take what are known as “world averages” when determining the masses of these particles. That is, we combine the different data sets, complete with errors and uncertainties, together, and then produce an estimate that combines them in a scientifically responsible fashion.
The best measurements for the mass of the top quark were released on March 19, 2024 jointly by the CMS and ATLAS collaborations at CERN, where they found a top mass of 172.52 ± 0.33 GeV: the highest-precision and lowest-uncertainty measurement of the top mass ever.
Meanwhile, the ATLAS collaboration has the lead over the CMS collaboration in determining the mass of the Higgs boson, finding, in a release from July of 2023, a Higgs mass of 125.11 ± 0.11 GeV, with a precision of just 0.09%: the greatest of all-time.
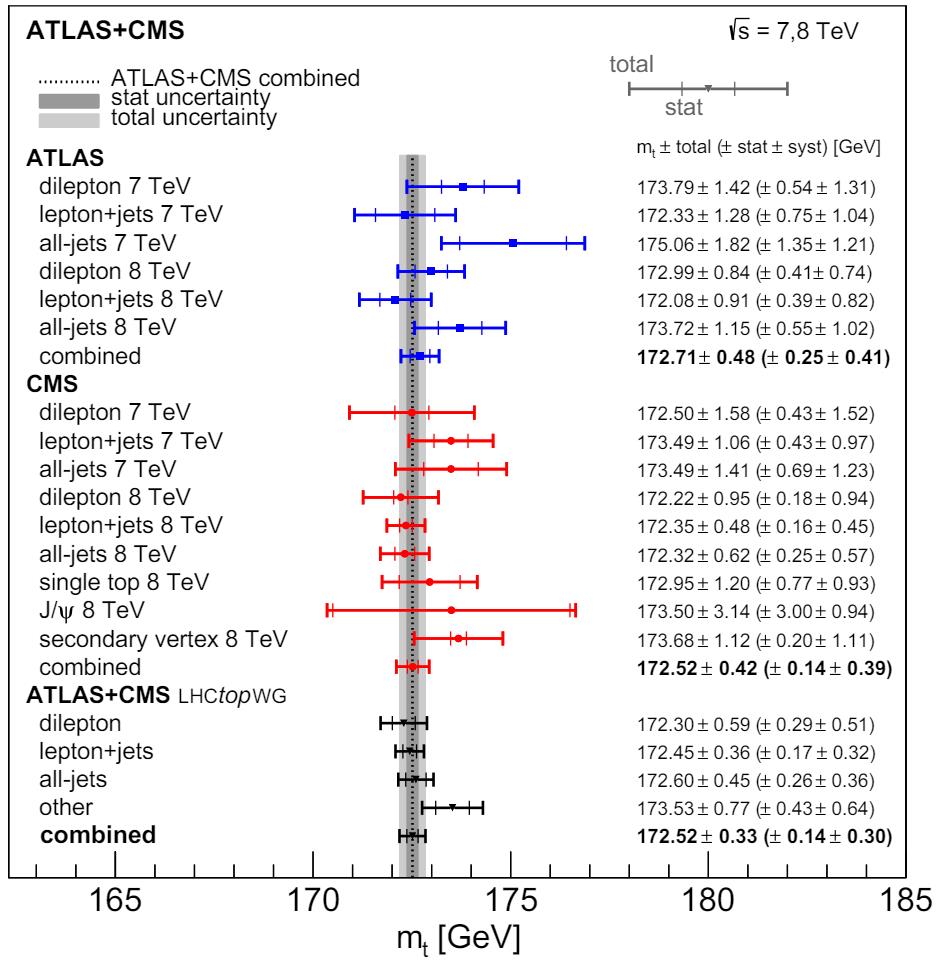
Possible masses and what they imply
If these measured masses are correct, then we would expect to see the W-boson with a specific mass dependent on which physical scenario describes our reality. With respect to supersymmetry, for instance, we’d expect to find the W-boson’s mass to be:
- around 80.36 GeV, if the Standard Model and no supersymmetry is what’s out there,
- around 80.38 GeV, if there is supersymmetry but the particles are heavy, perhaps too heavy to be seen at the LHC,
- or around 80.43 GeV, if there is supersymmetry and the supersymmetric particles are well within reach of the LHC.
The latter two scenarios are astounding, as any indication that there’s something out there beyond the Standard Model that can be found by colliders — whether direct or indirect — is a tantalizing possibility worth exploring. If the CDF result for the W-boson mass, of 80.433 GeV, is right, then it implies that we’re right on the doorstep of making a new discovery at the LHC. If the earlier results for the W-boson’s mass are correct, it implies that there is new physics out there to discover: perhaps out of reach of the LHC, but perhaps within reach of a next-generation collider. And, if everyone is wrong and it’s just a boring, plain-vanilla Universe out there, then we’d expect to see the W-boson’s mass regress to the Standard Model’s predictions: 80.36 GeV or thereabouts.
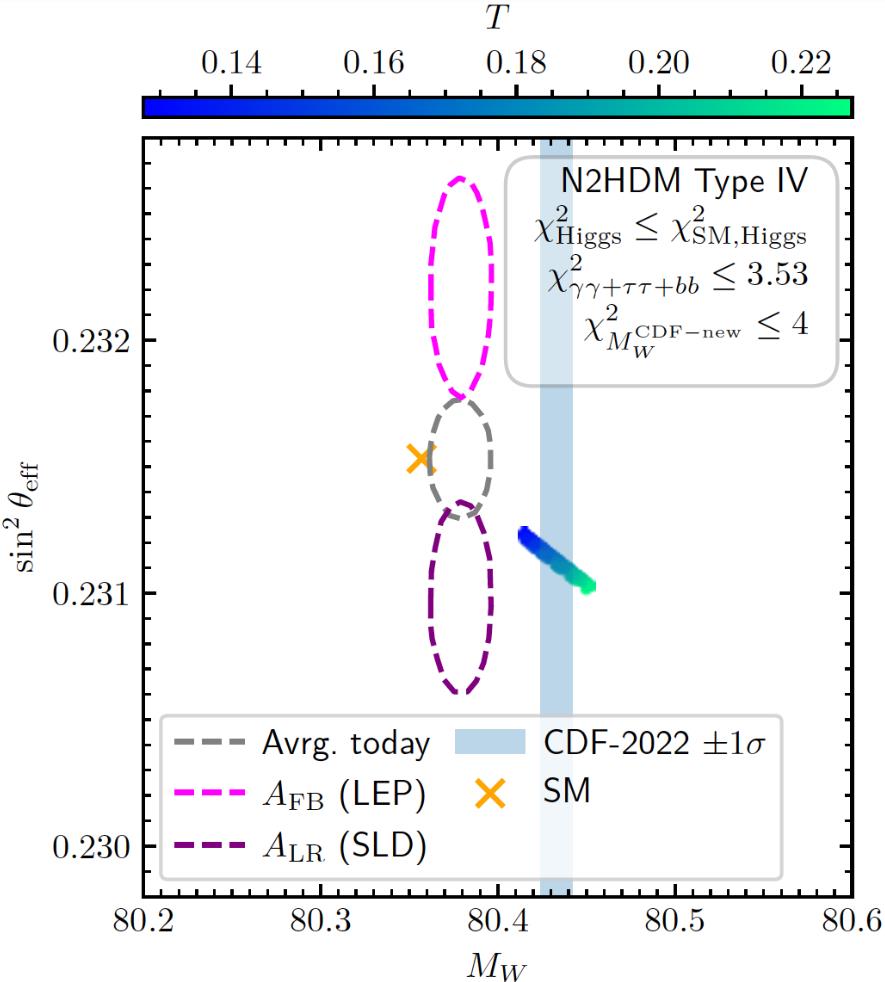
Fermilab vs. the LHC
It may seem bizarre, especially if you yourself aren’t an experimental physicist, to learn that the CDF collaboration from Fermilab has delivered higher-precision results, when it comes to measuring the mass of the W-boson, than either the CMS collaboration or the ATLAS collaboration has been able to deliver from CERN. Fermilab, after all, shut down for good on September 30, 2011, whereas the LHC has been operating off-and-on throughout the 13 subsequent years. The LHC has collected more data, has created more collisions, and has produced more W-bosons than Fermilab ever did.
So why are the error bars associated with the ATLAS collaboration larger than the CDF collaboration’s, and why doesn’t the CMS collaboration have any W-boson measurements at all? There are two main reasons.
- Fermilab’s Tevatron collided particles (protons and antiprotons) at much lower energies (by about a factor of seven) than CERN collides particles (protons and protons). At Fermilab, almost all of the W-bosons created are made from quark-antiquark collisions, and at lower energies, it’s relatively easy to model how the proton’s (or antiproton’s) energy is distributed among its quarks. However, at CERN, most W-bosons are created when a quark collides (through a “sea” quark) with a gluon, where the energy distribution, known as the parton distribution function, is much more difficult to model.
- Collisions at the LHC are much more frequent: about ten times as frequent, and also occur much closer together in time. This makes reconstructing what happens in any one collision more difficult at the LHC; you’re more likely to discover something novel, but precision work about the parameters of a short-lived particle, like the W-boson, is far more complex.
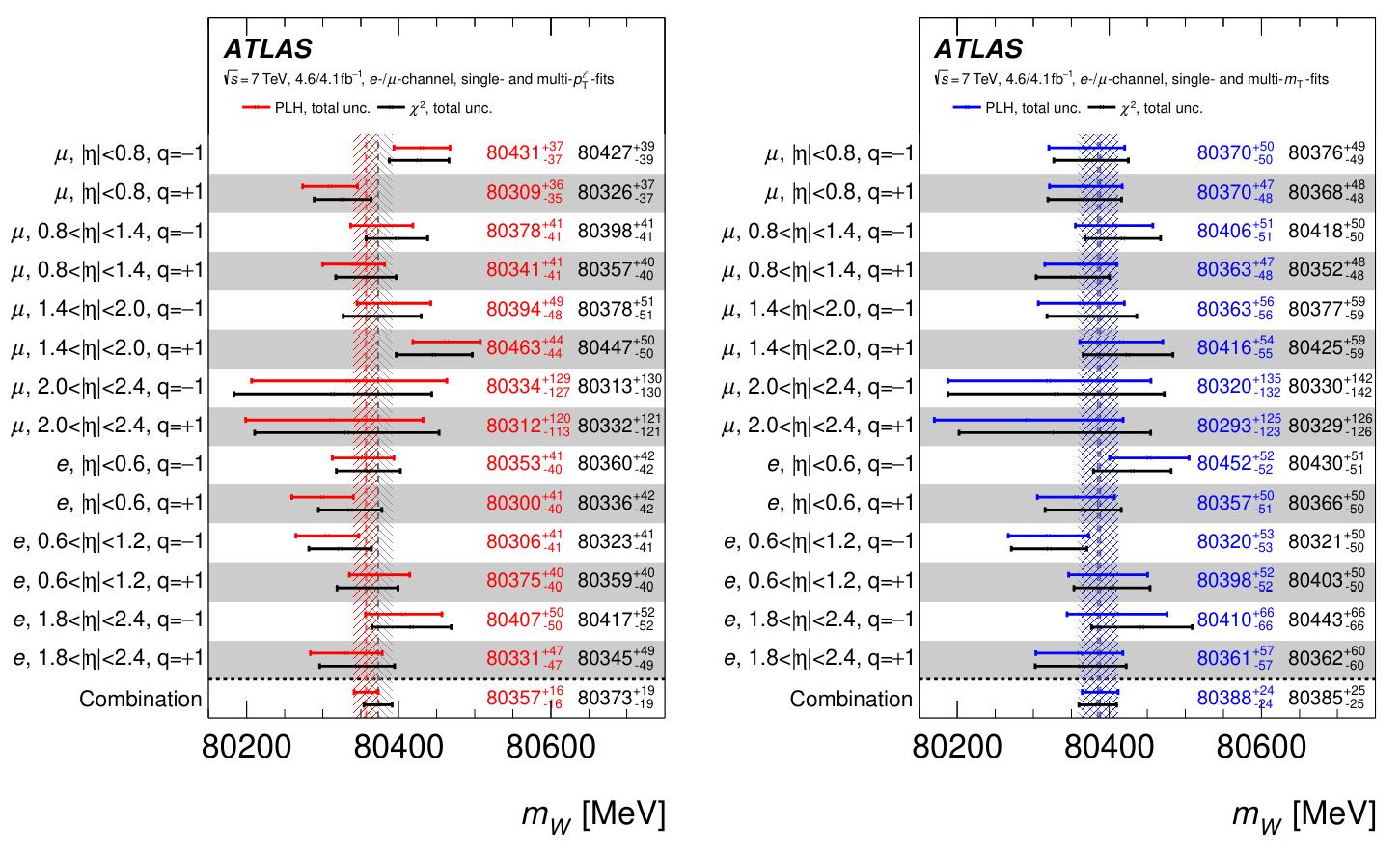
The latest results from ATLAS
Even though the CMS collaboration hasn’t quite gotten there (yet), the ATLAS collaboration — the other of the two main detectors at CERN’s Large Hadron Collider — has managed to make an improved determination of the W-boson mass, separated into different:
- decay modes (whether into an electron or a muon),
- by the charge of the original W-boson (positive or negative),
- and by how large its transverse momentum is relative to the beam direction (the parameter η, which means pseudorapidity in particle physics-speak).
When the data is combined together, cumulatively, ATLAS scientists were finally able to determine what their best estimate is for the mass of the W-boson, and what the (combined statistical and systematic) uncertainties are on that measured mass. Whereas the previous, pre-CDF world average from 2022 was 80.379 ± 0.012 GeV, and the CDF results were 80.433 ± 0.009 GeV, the new ATLAS results, published in March of 2024, indicate a new mass for the W-boson of 80.367 ± 0.016 GeV. The uncertainty may be nearly twice as large as the reported CDF uncertainties, but the two measurements are not mutually compatible; they exclude one another at about the 99.9% confidence level.
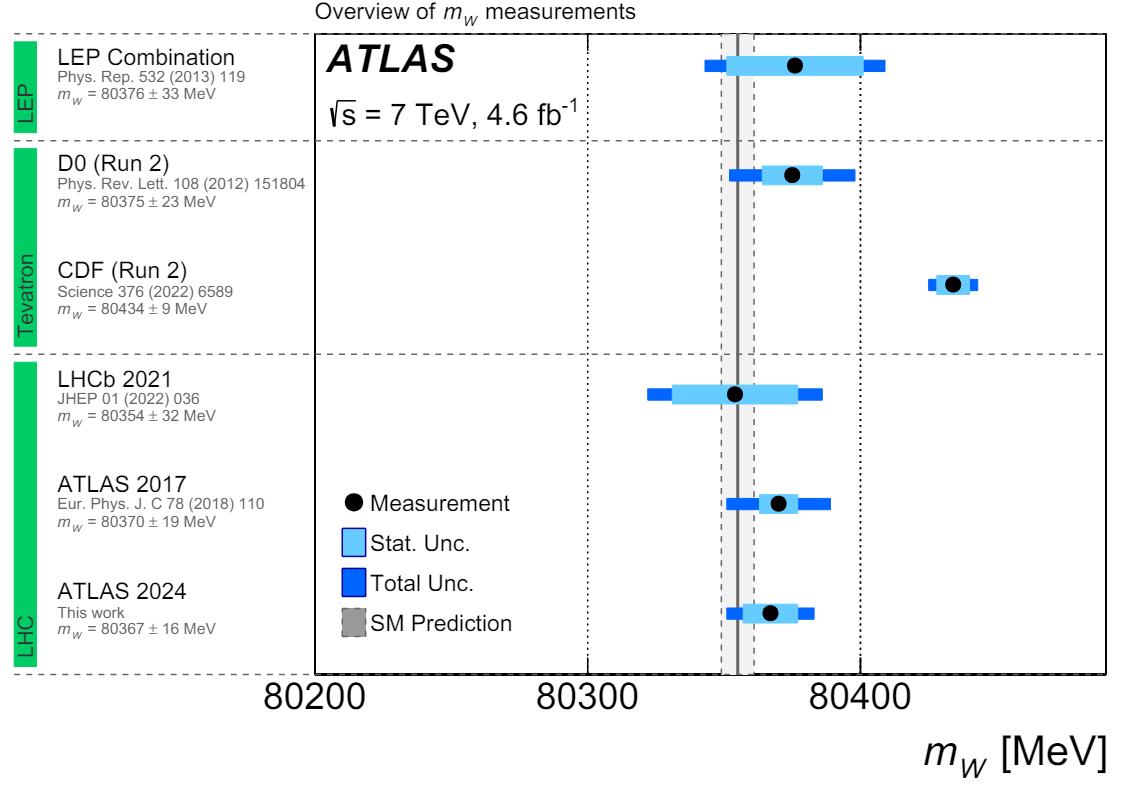
In fact, an independent group took the full suite of data available about the mass of the W-boson from four different collaborations (ATLAS, LHCb, CDF, and D0), and found that there’s less than a 1% chance that all of the measured data sets are compatible with one another. When the CDF measurement is removed, there’s all of a sudden a greater than 90% chance that the remaining data sets are compatible, and they use those to obtain a world average mass for the W-boson of 80.369 ± 0.013 GeV, which is again incompatible at the ~99.9% level with the data from the CDF collaboration.
Presumably, there was an error made at some point along the line in the CDF analysis. Ideally, someone would be able to go through the CDF analysis and successfully find wherever they made that error, which should then bring all the various measurements from different collaborations into alignment. But that has not come to pass. Instead, we are only left with the various assertions of different groups, and are left with no option but to discard the CDF data as an outlier that sticks out from all other measurements. When the statistics improve with greater amounts of data from ATLAS, and when the CMS collaboration finally uses their data (they have everything they need) to put forth an estimate for the W-boson mass, we should see if that approach is justified, and if they yield a mass value that’s consistent with the Standard Model alone.

Has the Standard Model survived, or is it broken?
Ultimately, this is the key question we need to ask ourselves: has the Standard Model survived this test? Sure, there are places where the Standard Model is insufficient: as respects dark matter, dark energy, the origin of the matter-antimatter asymmetry, the lack of observed strong CP violation, and the origin of neutrino mass, for starters. But for the properties of the W-boson itself, we need look only at the above graph from the linked ATLAS paper. (I’ve added in a red “star” to indicate the precise Standard Model, alone, prediction.) Based on the mass of the top quark (dark blue line/x-axis) and the properties of the Higgs boson (grey line and contours), the W-mass should be 80.357 GeV, and the measured value by ATLAS, of 80.367 ± 0.016 GeV, is in perfect agreement with that prediction.
It’s worth noting that you can’t simply take more data and decrease those errors; about 0.0125 GeV of those uncertainties, according to the ATLAS collaboration themselves, comes from systematic errors, or errors inherent to performing the experiment itself. That is the big challenge ahead for those of us hoping to test, at the hundredth-of-a-percent level, whether the masses of the Higgs boson, the top quark, and the W-boson are all consistent with the Standard Model (if they meet at the “star” in the diagram above), or whether there truly is an indication that new physics is right around the corner, just waiting to be discovered. It’s the ultimate detective story, and thousands of the best physicists in the world are on the case!