Why Johannes Kepler is a scientist’s best role model
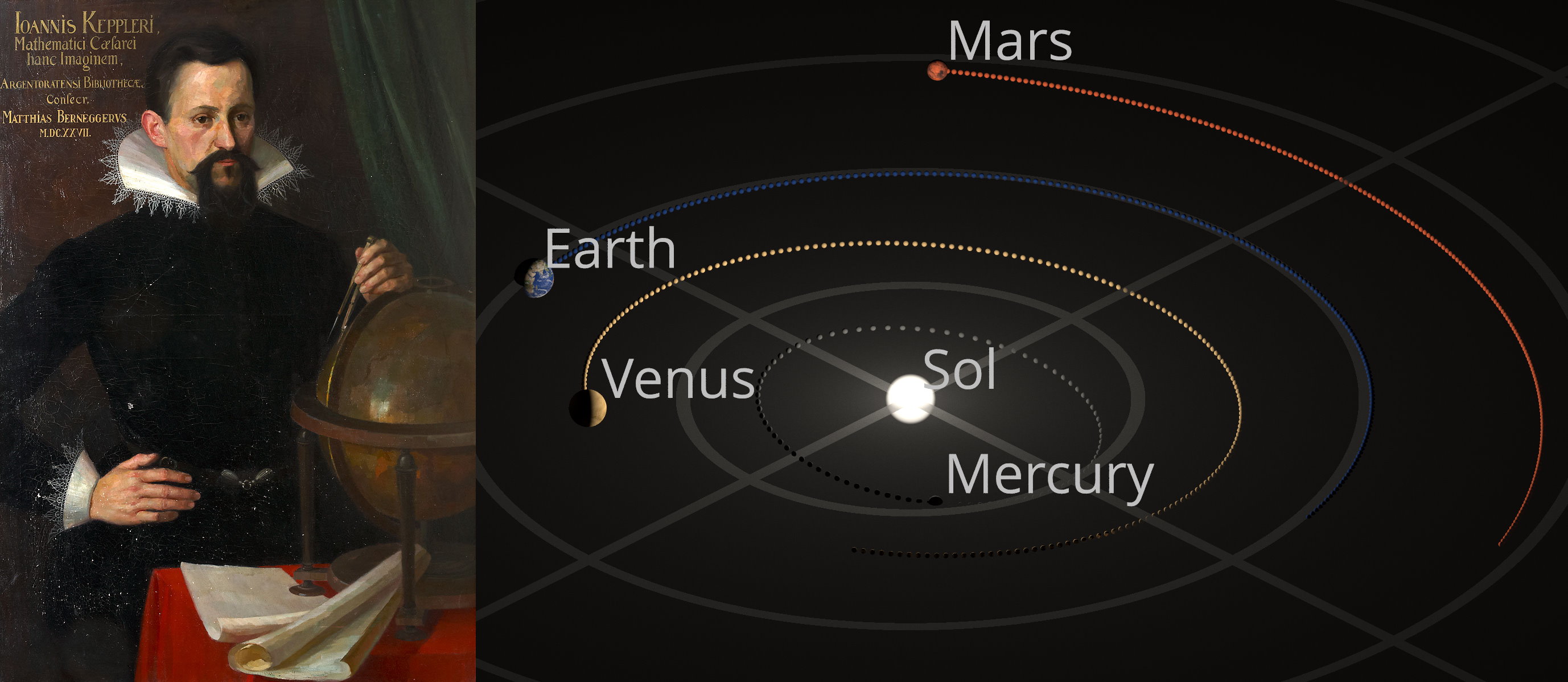
- The annals of history are filled with scientists who had incredible, revolutionary ideas, sought out and found the evidence to support them, and initiated a scientific revolution.
- But much rarer is someone who has a brilliant idea, discovers that the evidence doesn't quite fit, and instead of doggedly pursuing it, tosses it aside in favor of a newer, better, more successful idea.
- That's exactly what separates Johannes Kepler from all of the other great scientists throughout history, and why, if we have to choose a scientific role model, we should admire him so thoroughly.
For a great many people in the world, the three hardest words to say are simply, “I was wrong.” Even if the evidence is overwhelmingly decisive that your idea or conception is unsupported, most people will instead find a way to discount or ignore that evidence and stick to their guns. People’s minds are notoriously resistant to change, and the greater their own personal stake in the outcome of the issue under debate, the less open they are to even the possibility that they might be mistaken.
Although it’s often asserted that science is the exception to this general rule, that’s only true of science as a collective enterprise. On an individual basis, scientists are just as susceptible to confirmation bias — overweighting the supporting evidence and discounting the evidence to the contrary — as anyone in any other walk of life. In particular, the greatest difficulties await those who themselves have formulated ideas and invested tremendous efforts, often amounting to years or even decades of time, in hypotheses that simply cannot explain the full suite of data that humanity has amassed. This applies even to the greatest minds in all of history.
- Albert Einstein could never accept quantum indeterminism as a fundamental property of nature.
- Arthur Eddington could never accept quantum degeneracy as a source for holding white dwarfs up against gravitational collapse.
- Newton could never accept the experiments that demonstrated the wave nature of light, including interference and diffraction.
- And Fred Hoyle could never accept the Big Bang as the correct story of our cosmic origins, even nearly 40 years after the critical evidence, in the form of the Cosmic Microwave Background, was discovered.
But one person stands above the rest as an exemplar for how to behave when the evidence comes in against your brilliant idea: Johannes Kepler, who showed us the way more than 400 years ago. Here’s the story of his scientific evolution, an example we should all strive to emulate.
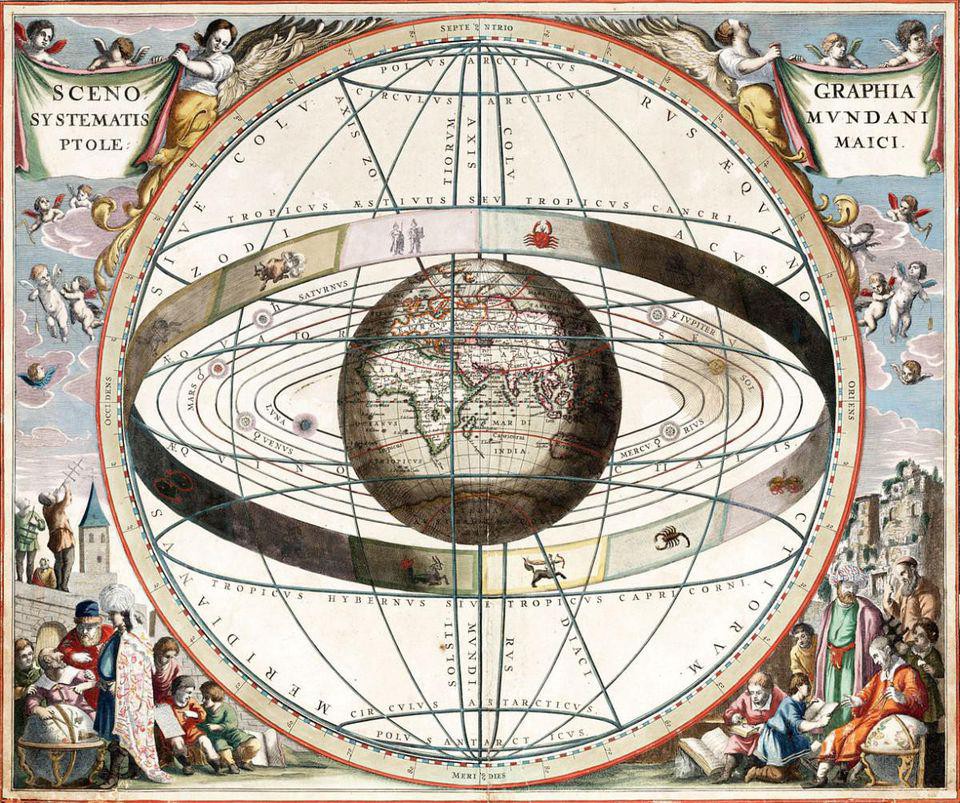
For thousands of years, humans assumed that the Earth was a static, stable, and unchanging point in the Universe, and that all the heavens literally moved around us. Observations seemed to support this: there was no detectable motion occurring on our surface that supported an Earth that either rotated on its axis or revolved around the Sun through space. Instead, there were three key observations that had been made that helped people determine what our best model of the Universe would be.
- The entire sky appeared to rotate a full 360 degrees over the course of 24 hours, most evident at night, as the stars rotated about either the northern or southern celestial pole.
- The stars themselves appeared to remain fixed in their relative position to one another from night-to-night and even over much longer timescales.
- However, there were a few objects that did move relative to one another from night-to-night or day-to-day: the planets, or “wanderers” of the sky.
Additionally, the Sun and Moon shifted in the night as well, as did the entire canopy of stars over longer periods of time. However, it was the first observation that led to the static, stable, unchanging conception of the Universe.
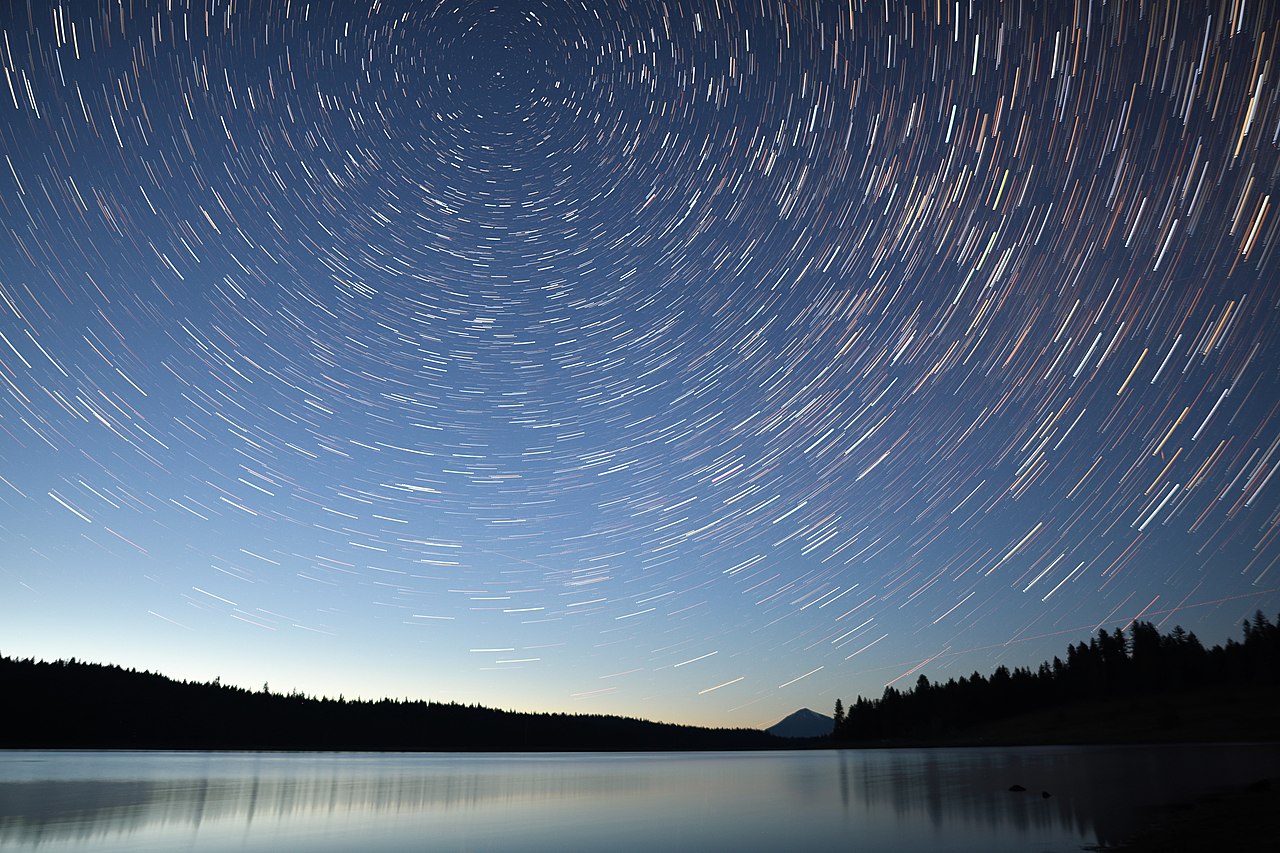
Think about the above observation: that everything in the sky appears to rotate a full 360 degrees over the span of a full day. This could be caused by one of two potential explanations. Either the Earth itself was rotating about some axis, and that our world completed a full rotation once per 24 hours, or the Earth was stationary and everything in the heavens was rotating around it, also once per 24 hours.
How, physically, could we tell these two situations apart? The answers were twofold.
First, it should be possible, if the Earth were rotating, to note a curved trajectory to falling objects. The higher they fell from, the greater the curve would be. Yet no curve was ever observed; in fact this effect wouldn’t be measured until the demonstration of the Foucault pendulum in the 19th century.
Second, a rotating Earth would lead to a difference in the relative positions of the stars from dusk until dawn. The Earth was big, and its diameter had been measured precisely by Eratosthenes in the 3rd century B.C.E., so if any of the stars were closer than most of them, a parallax would appear: similar to holding your thumb out and watching it shift relative to the background as you alternated which eye you used to view it. But no parallax could be seen; in fact this wouldn’t be observed until the 19th century as well!

It’s easy to see, based on what we knew and could observe at the time, how we’d conclude that the Earth was static and fixed, while the heavenly bodies all moved around us.
Then, there were those additional observations that required an explanation: why did the stars remain fixed relative to one another while the planets appeared to “wander” through the sky?
It was quickly modeled that the planets, as well as the Sun and the Moon, must be closer to Earth than the stars were, and that these bodies must be in motion relative to one another.
With a fixed, static Earth, that meant that it must be the planets themselves that were in motion. The motion must have been incredibly complex, however. While the planets overwhelmingly appeared to move in one direction relative to the backdrop of stars on a night-to-night basis, every once in a while, the planets would:
- slow down in their usual motion,
- come to a complete stop,
- reverse their motion to move opposite their original direction (a phenomenon known as retrograde motion),
- would then slow and stop again,
- and finally would continue on in their normal (prograde) direction of motion.
This phenomenon was the most challenging aspect of planetary motion to model and understand.
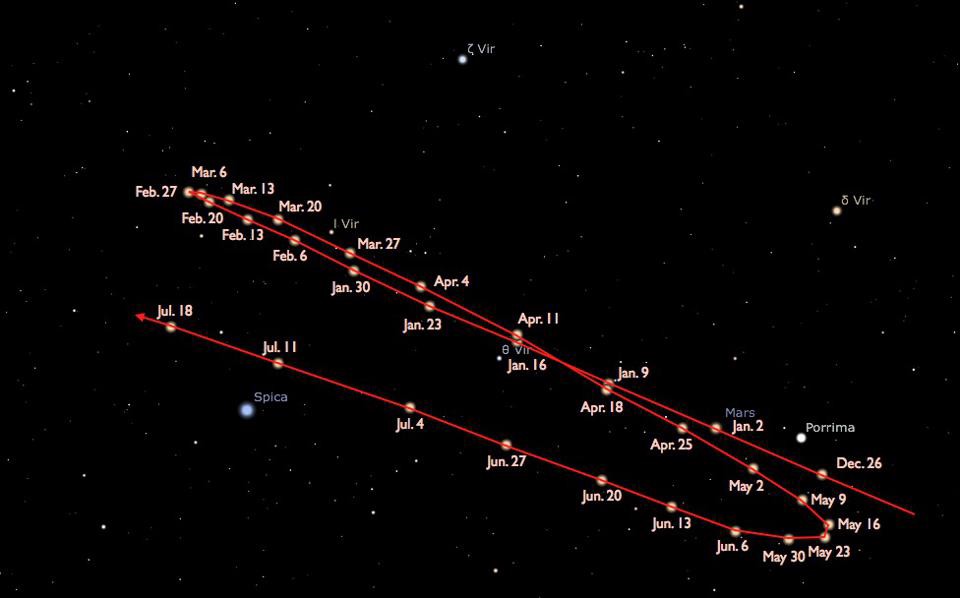
The prevailing assumption, since the Earth had already been deemed to be static, was that the planets themselves each typically moved in circular paths around the Earth, but atop those circles were smaller circles known as “epicycles” that they moved about as well. When the motion through the smaller circle proceeded in the opposite direction from the main motion through the larger circle, the planet would appear to reverse course for a brief while: a period of retrograde motion. Once the two motions lined up in the same direction again, prograde motion would resume.
Although epicycles did not start with Ptolemy — with whose name they are now synonymous — Ptolemy did make the best, most successful model of the Solar System that incorporated epicycles. In his model, the following occurred.
- Each planet’s orbit was dominated by a “great circle” that it moved along, moving around the Earth.
- Atop each great circle, a smaller circle (an epicycle) existed, with the planet moving along the outskirts of that small circle, with the center of the small circle always moving along the larger one.
- And the Earth, rather than being at the center of the great circle, was offset from that center by a particular amount, with the specific amount differing for each planet.
That was the Ptolemaic theory of epicyclic motion, leading to a geocentric model of the Solar System.
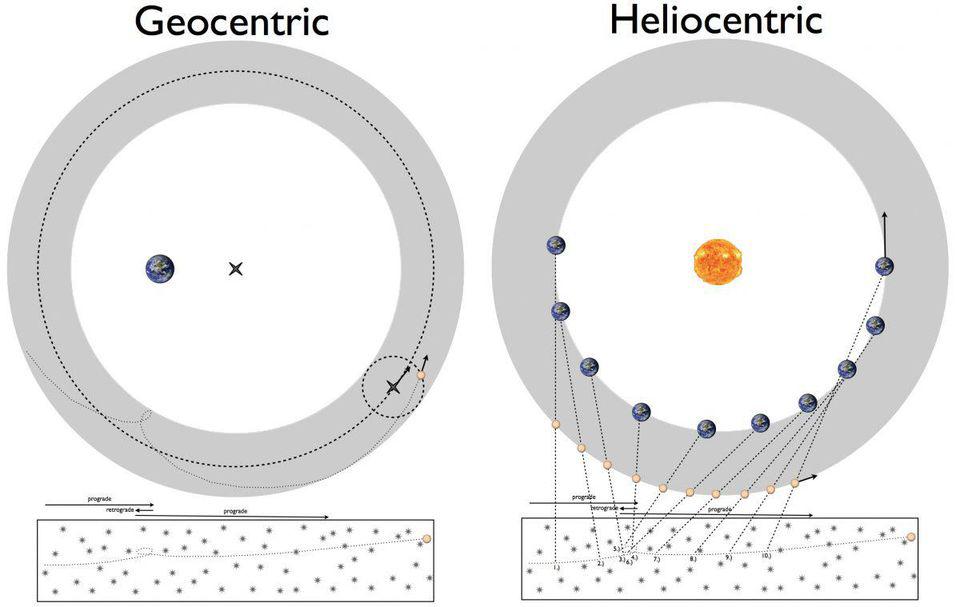
Going all the way back to ancient times, there was some evidence — from Archimedes and Aristarchus, among others — that a Sun-centered model for planetary motion was considered. But once again, the lack of any detectable motion for the Earth or of any detectable parallax for the stars failed to provide the corroborating evidence. The idea languished in obscurity for centuries, but was finally revived in the 16th century by Nicolaus Copernicus.
The great idea of Copernicus was that if the planets moved in circles around the Sun, then during most times, the inner planets would orbit more quickly than the outer ones. From the perspective of any one planet, the others would appear to migrate relative to the fixed stars. But whenever an inner planet passed by and overtook an outer planet, then retrograde motion would occur, as the normal apparent direction-of-motion would appear to reverse.
Copernicus realized this and put forth his theory of a Sun-centered Solar System, or a heliocentric (rather than geocentric) one, offering it up as an exciting and possibly superior alternative to Ptolemy’s older Earth-centered model.

But in science, we always have to follow the evidence, even if we loathe the path it leads us down. It’s not aesthetics, elegance, naturalness, or personal preference that decides the issue, but rather the success of the model in predicting what can be observed. Leveraging circular orbits for both the Ptolemaic and the Copernican models, Copernicus was frustrated to discover that his model gave less successful predictions when compared against Ptolemy’s. The only way Copernicus could devise to equal Ptolemy’s successes, in fact, relied on employing the same ad hoc fix: by adding epicycles, or small circles, atop his planetary orbits!
In the decades following Copernicus, others took interest in the Solar System. Tycho Brahe, for example, constructed the best naked eye astronomy setup in history, measuring the planets as precisely as human vision allows: to within one arc-minute (1/60th of a degree) during every night that planets were visible towards the end of the 1500s. His assistant, Johannes Kepler, attempted to make a glorious, beautiful model that fit the data precisely.
Given that there were six known planets (if you included the Earth as one of them), and exactly five (and only five) perfect polyhedral solids — the tetrahedron, cube, octahedron, icosahedron, and dodecahedron — Kepler constructed a system of nested spheres called the Mysterium Cosmographicum.
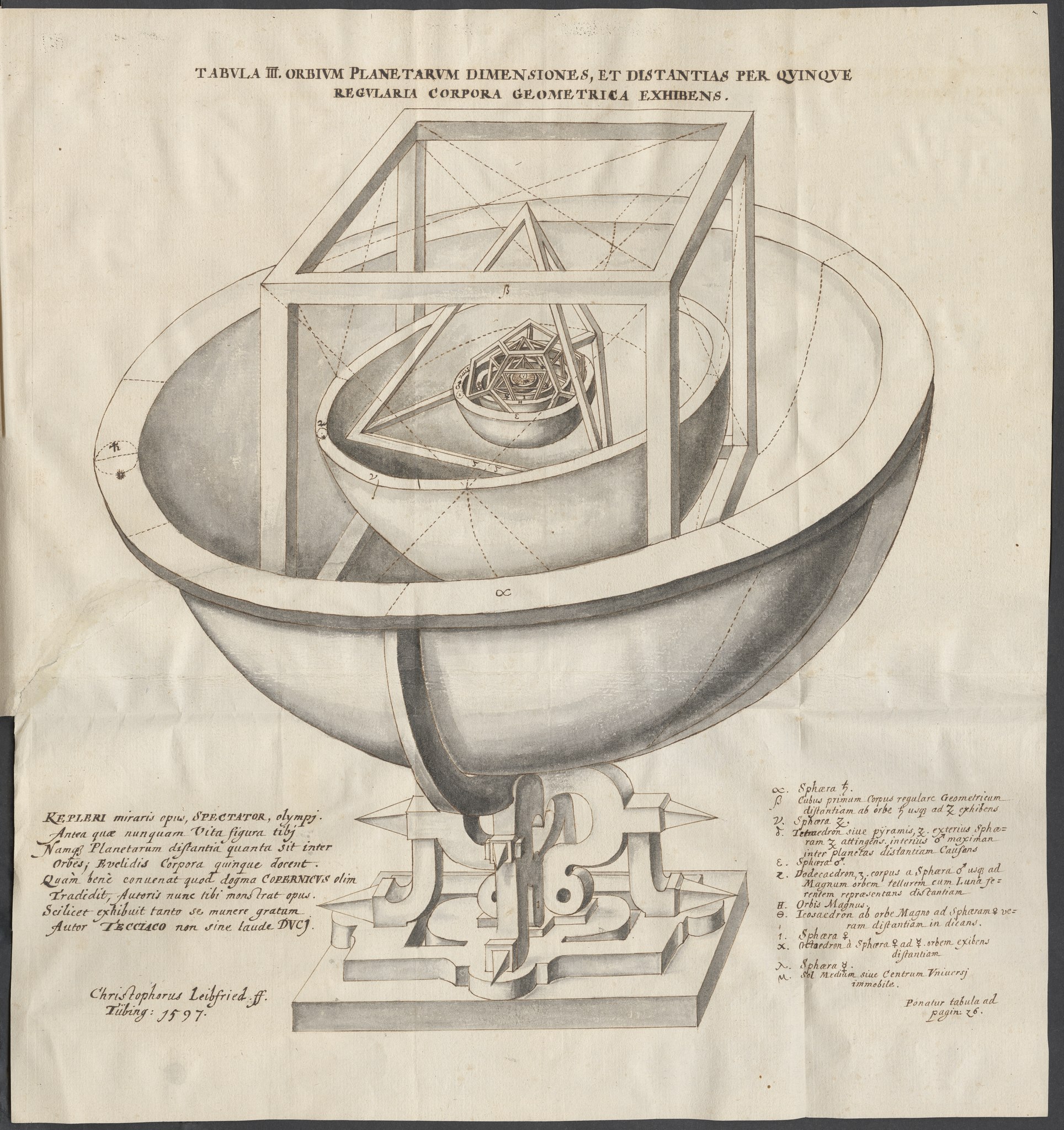
In this model, each planet orbited along a circle defined by the circumference of one of the spheres. Outside of it, one of the five Platonic solids was circumscribed, with the sphere touching each of the faces in one spot. Outside of that solid, another sphere was circumscribed, with the sphere touching each of the solid’s vertices, with the circumference of that sphere defining the orbit of the next planet out. With six spheres, six planets, and five solids, Kepler made this model where “invisible spheres” held up the Solar System, accounting for the orbits of each of Mercury, Venus, Earth, Mars, Jupiter, and Saturn.
Kepler formulated this model in the 1590s, and Brahe boasted that only his observations could put such a model to the test. But no matter how Kepler did his calculations, not only did disagreements with observation remain, but Ptolemy’s geocentric model still made superior predictions.
In the face of this, what do you think Kepler did?
- Did he tweak his model, attempting to save it?
- Did he distrust the critical observations, demanding new, superior ones?
- Did he make additional postulates that could explain what was truly occurring, even if it was unseen, in the context of his model?
No. Kepler did none of these. Instead, he did something revolutionary: he put his own ideas and his own favored model aside, and looked at the data to see if there was a better explanation that could be derived from demanding that any model needed to agree with the full suite of observational data.
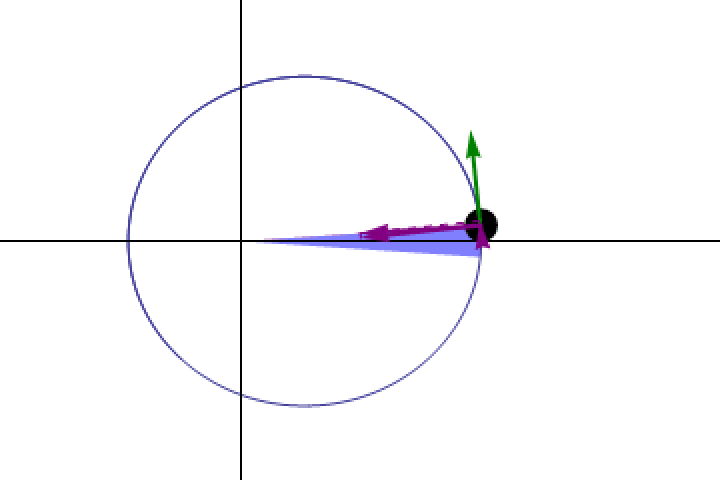
If only we could all be so brave, so brilliant, and at the same time, so humble before the Universe itself! Kepler calculated that ellipses, not circles, would better fit the data that Brahe had so painstakingly acquired. Although it defied his intuition, his common sense, and even his personal preferences for how he felt the Universe ought to have behaved — indeed, he thought that the Mysterium Cosmographicum was a divine epiphany that had revealed God’s geometrical plan for the Universe to him — Kepler was successfully able to abandon his notion of “circles and spheres” and instead used what seemed to him to be an imperfect solution: ellipses.
It cannot be emphasized enough what an achievement this is for science. Yes, there are many reasons to be critical of Kepler. He continued to promote his Mysterium Cosmographicum even though it was clear ellipses fit the data better. He continued to mix astronomy with astrology, becoming the most famous astrologer of his time. And he continued the long tradition of apologetics: claiming that ancient texts meant the opposite of what they said in order to reconcile the acceptability of the new knowledge that had emerged.
But it was through this revolutionary action, of abandoning his model for a new one that he himself devised to explain the observations more successfully than ever before, that Kepler’s laws of motion became elevated to scientific canon.

Even today, more than four full centuries after Kepler, we all learn his three laws of planetary motion in schools.
- Planets move in ellipses around the Sun, with the Sun at one of the ellipse’s two focal points.
- Planets sweep out equal areas, with the Sun at once focus, in equal amounts of time.
- And planets orbit in time periods proportional to their semimajor axes (half of the longest-axis of the ellipse) to the 3/2 power.
These were the first calculations that advanced the science of astronomy beyond the stagnated realm of Ptolemy, and they paved the way for Newton’s theory of universal gravitation, which transformed these laws from simple descriptions of how motion occurred to one that was physically motivated. By the end of the 17th century, all of Kepler’s laws could be derived simply from the laws of Newtonian gravity.
But the greatest achievement of all was the day Kepler put his own idea of a Mysterium Cosmographicum — an idea that he was arguably more emotionally attached to than any other — in order to follow the data, wherever it led him. That brought him to elliptical orbits for the planets, which kicked off the revolution in our understanding the physical universe around us, i.e., the modern sciences of physics and astronomy, that continues to the present day. Like all scientific heroes, Kepler certainly had his faults, but the ability to admit when you’re wrong, to reject your insufficient ideas, and to follow the data wherever it leads are traits we should all aspire to. Not only in science, of course, but in all aspects of our lives.