Ask Ethan: Could gravity operate in extra dimensions?
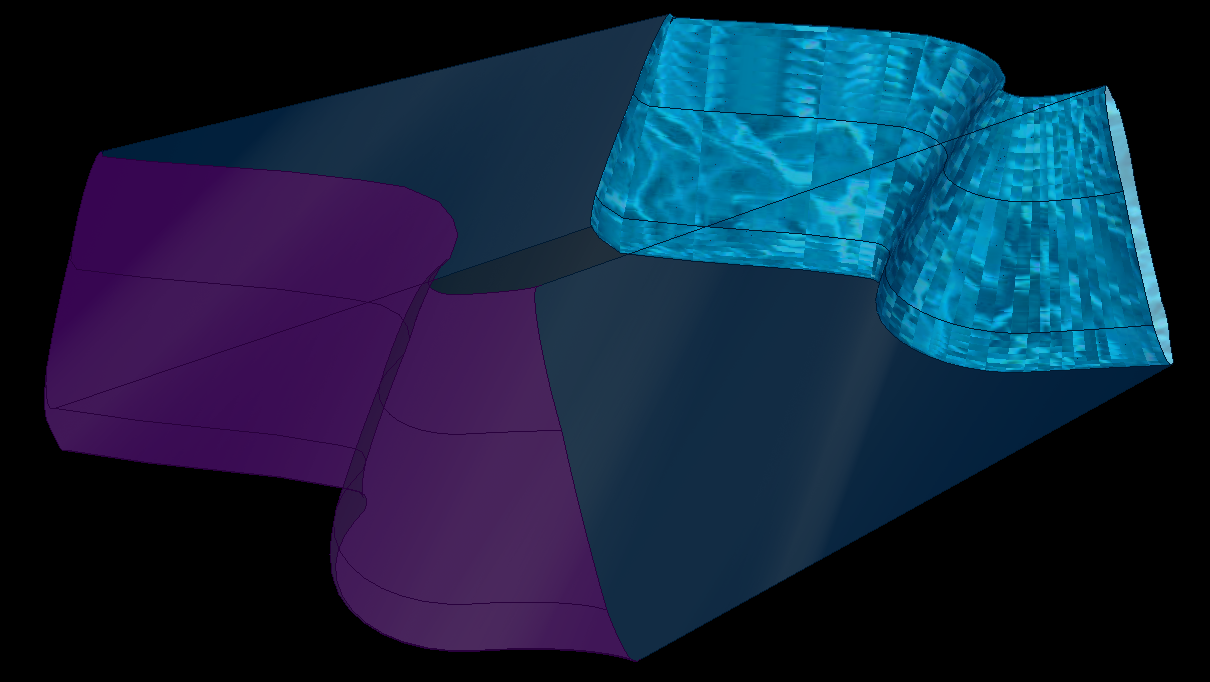
- Ever since the strengths of the four fundamental forces were first quantified, physicists have wondered why gravitation is so much fundamentally weaker than all the others.
- In the late 1990s, a radical proposal suggested that one possible reason is that, on very tiny scales, gravity could be “bleeding” into additional, extra dimensions, further weakening it on large scales.
- It’s an idea that’s absolutely worth exploring, but one that hasn’t had any evidence weigh in its favor so far. Here’s what everyone ought to know.
There’s a problem with gravity that no one is comfortable with and that even physicists rarely talk about. If you take any two particles with a mass to them — like two electrons, two of the quarks within a proton or neutron, or even composite particles like two protons — you’ll be able to calculate the strength of all four of the fundamental forces between them. When you do those calculations for the strong and weak nuclear forces, the electromagnetic force, and gravity, you’ll discover something that may puzzle you: the force of gravity, particularly at small distances, is far, far weaker than any of the other forces. Inside a neutron, for example, the force of gravity is more than 30 orders of magnitude (a factor of ~1030) weaker than each of the other three fundamental forces.
Why is that? Although nobody knows, one remarkable proposal put forth back in 1998 suggested that, perhaps, gravity is so weak because it, unlike the other forces, may be “bleeding” into extra dimensions at very small distances. That’s the scenario that Curtis “Ovid” Poe wants us to consider this week, asking:
“I read a proposal that gravity is perhaps so weak compared to the other forces because unlike them, it operates in more dimensions… If that’s possible, could it be that dark matter is matter outside our ordinary three (apparent) physical dimensions and that’s why we detect the gravity, but not the matter?”
It’s an interesting thing to consider: what could be hiding in these extra dimensions, if there really are any? Let’s find out.
I want you to start not by considering a force or higher-dimensional space, but rather something far simpler: the light coming from a star. As you move farther and farther away from that star, the light that was emitted radiates outward, away from it, in a shape known as a spherical shell. As you increase the distance between yourself and the star, you’ll find that the apparent brightness of the light source (the star) that you observe appears to drop off as the square of the distance: one-quarter of the original brightness for each doubling of the distance. This is because:
- the light spreads out spherically,
- the surface area of a sphere of radius R is 4πR²,
- and so as the light propagates outward to greater distances, its observed brightness drops off proportional to ~1/R².
It’s easy and intuitive to see, and you can envision forces like gravitation and electromagnetism this way as well: that the farther away you are from a source, like a massive or an electrically charged particle, the less strongly that interaction affects you. The force of gravity between any two masses falls off as ~1/R², the electric force between two charged particles falls off as ~1/R², and — just like the light spreading out in a spherical shape as it leaves a source — you can imagine that the strength of the interaction falls off in exactly the same way.

But here’s a hypothetical question to ponder: how would all three of these phenomena — the way that light spreads out, the way that the force of gravity falls off, or the way that the electromagnetic force falls off — vary with distance if we didn’t live in a Universe with three spatial dimensions?
We can actually test this out, experimentally, in many condensed matter (materials-based) systems that confine certain phenomena to either a surface (which is fundamentally two-dimensional) or even a line-or-cable (which is fundamentally one-dimensional) to see how this sort of spreading-out works. Instead of a three-dimensional sphere, things could only spread out in
- either a circle (for the two-dimensional case),
- or confined to that same “point” as it propagates down the line (for the one-dimensional case),
which means that instead of falling off as ~1/R² (which was the three dimensional case), a two-dimensional system will only fall off as ~1/R (as the circumference of a spreading-out circle is just 2πR), while a one-dimensional system will remain constant, as there’s no “direction” where spreading-out at all.
Although it’s easiest to implement with light (such as in a one-dimensional fiber optic cable), there are many physical effects and phenomena that can be confined to two or one dimensions, rather than allowing them to propagate freely through all three spatial dimensions.
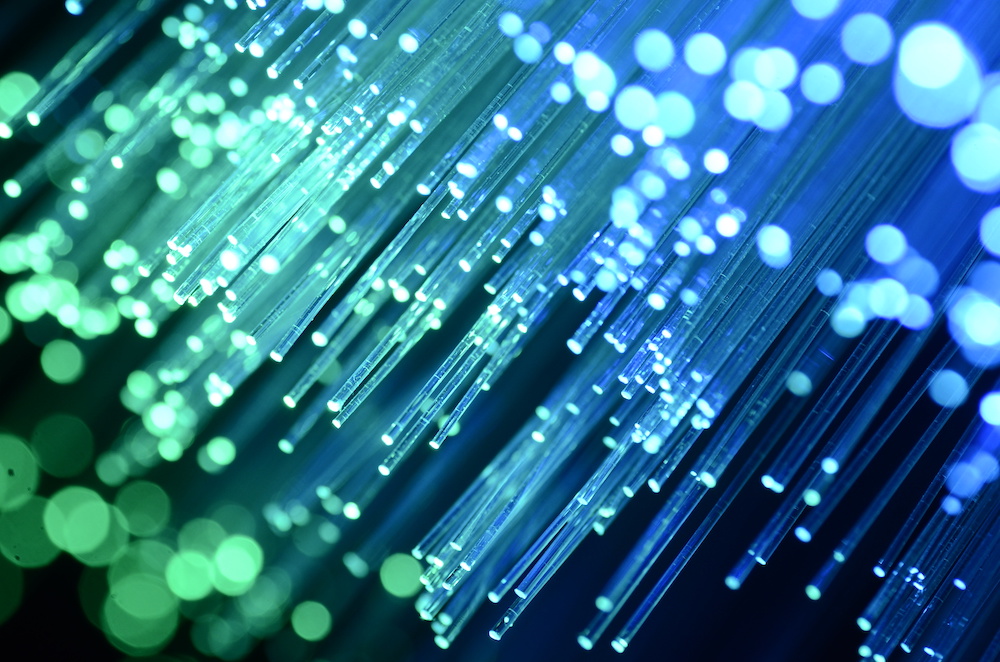
With fewer dimensions to propagate through — or, alternatively to “spread out” in — signals get weaker more slowly with increasing distance. One example to consider is a light source embedded in a pane of glass, where the light can propagate throughout that pane of glass, but cannot escape it. This pane of glass is going to be large in two dimensions (e.g., the length and width dimensions), but small in the third dimension (e.g., the depth dimension).
If you’re an observer who’s close to the light source — in other words, an observer whose distance to the light source is small compared to the depth of the third dimension — that light is going to spread out like a sphere for you, meaning you’ll see the brightness fall off as it would for a three-dimensional source: like ~1/R².
However, if you’re an observer who’s far away from the light source — in other words, an observer whose distance from the light source is large compared to the depth of the third dimension but still small compared to the extent of the other two (length and width) dimensions — then that light is going to:
- spread out like a sphere for the first part of its journey (falling off as ~1/R²), until it reaches the end of the “depth” dimension,
- and then spread out like a circle for the remaining part of its journey, with the brightness of the light thereafter only falling off as ~1/R.
When you’re close to the source, its brightness falls off more quickly, as it “sees” and can spread out within a greater number of dimensions, but when you’re farther away, its brightness falls off more slowly, as it “sees” and spreads out within a smaller number of dimensions.
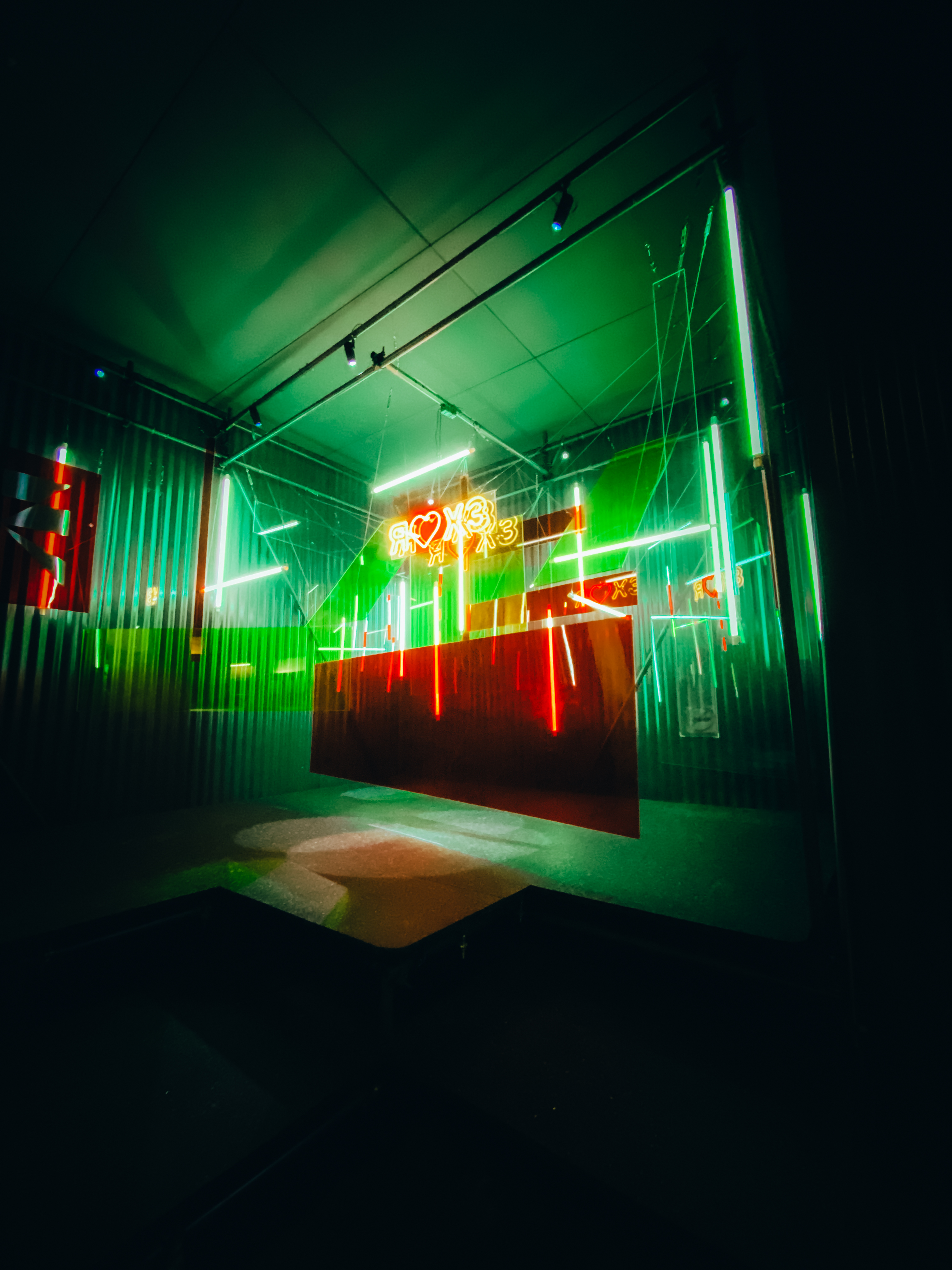
Think about this for a minute: in greater numbers of dimensions, signals fall off (or weaken) very rapidly, while in smaller numbers of dimensions, signals fall off (or weaken) more slowly, all the way down to a limit of “one-dimensional” where things don’t fall off or weaken at all.
Once you’ve gotten that down, you might start to ask, “Okay, well what does that have to do with explaining why gravitation is, as a force, so much weaker than all of the other fundamental forces?”
That’s where the idea of Large Extra Dimensions comes in. Initially proposed in 1998 by the team of Nima Arkani-Hamed, Savas Dimopoulos, and Gia Dvali (where it’s sometimes known as the “ADD” model, after the last names of the authors), it postulated that perhaps there truly are additional spatial dimensions out there — whether one or more than one — and that at least one of them may be large in physical extent. (Large, in this context, just means “large compared to the Planck scale, of ~10-35 meters,” not “macroscopically large.”)
If so, it’s possible that while the other three fundamental forces are confined to “spreading out” only in our conventional three spatial dimensions, the force of gravity might, below the distance scale of the large extra dimension(s), spread out in not only the conventional three but also any additional large extra dimensions.

Before you object, consider that in 1998, we had experimental evidence for the behavior of the three quantum forces down to the scales probed by particle colliders such as Fermilab and LEP, to scales of about ~10-18 meters, showing that they “spread out” in only three dimensions down to that tiny distance scale. (In the subsequent time since, the Large Hadron Collider at CERN has improved those constraints by adding an extra significant figure: down to ~10-19 meters.) If there were any extra dimensions that these three forces — the strong, weak, and electromagnetic forces — experienced, they could only exist at distance scales smaller than these.
But for gravitation, being such an incredibly weak force, it’s very challenging to detect gravitational effects down to similarly small scales. In fact, in 1998, we had only probed the nature of the force of gravity in laboratory experiments down to ~millimeter (~10-3 meter) scales! In other words, there were 15 orders of magnitude to play with — from millimeter scales down to attometer scales — where if there were one or more extra dimensions where gravity (but not the other forces) could “spread out” in, that could be the explanation for the 30+ orders of magnitude difference we see today, in our macroscopic world, between the strength of the gravitational interaction and all the others.
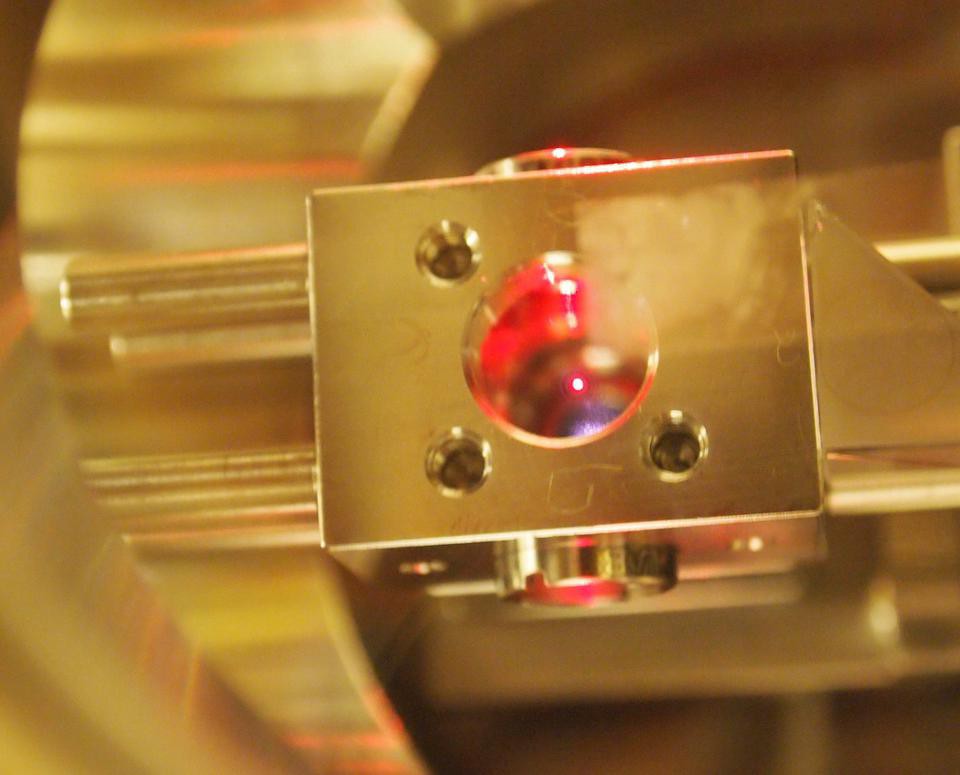
But things wouldn’t remain in that open-ended state for long. A new slate of experiments, including those using microscopic force probes and optically levitated microspheres in a vacuum, were able to show that gravitation obeyed the Newtonian-like ~1/R² force law down to micron-sized scales, demonstrating that there’s no “leaking” or “spreading out” of the gravitational force into extra dimensions down to those smaller scales.
However, there are pathologies that one has to worry about. If there are large extra dimensions out there in nature — and remember, large just means bigger than the Planck scale, or ~10-35 meters — they would lead to the production of microscopic quantum black holes (or Kaluza-Klein gravitons) at powerful enough particle colliders; the lack of any such signal at the LHC has constrained the energy scale of any plausible extra dimensions to be above about ~6 TeV.
Independently, from gamma-ray observations of neutron stars, such as with NASA’s Fermi-LAT instrument, we can further constrain any extra-dimensional scenarios to exclude any scenario with just one large extra dimension.
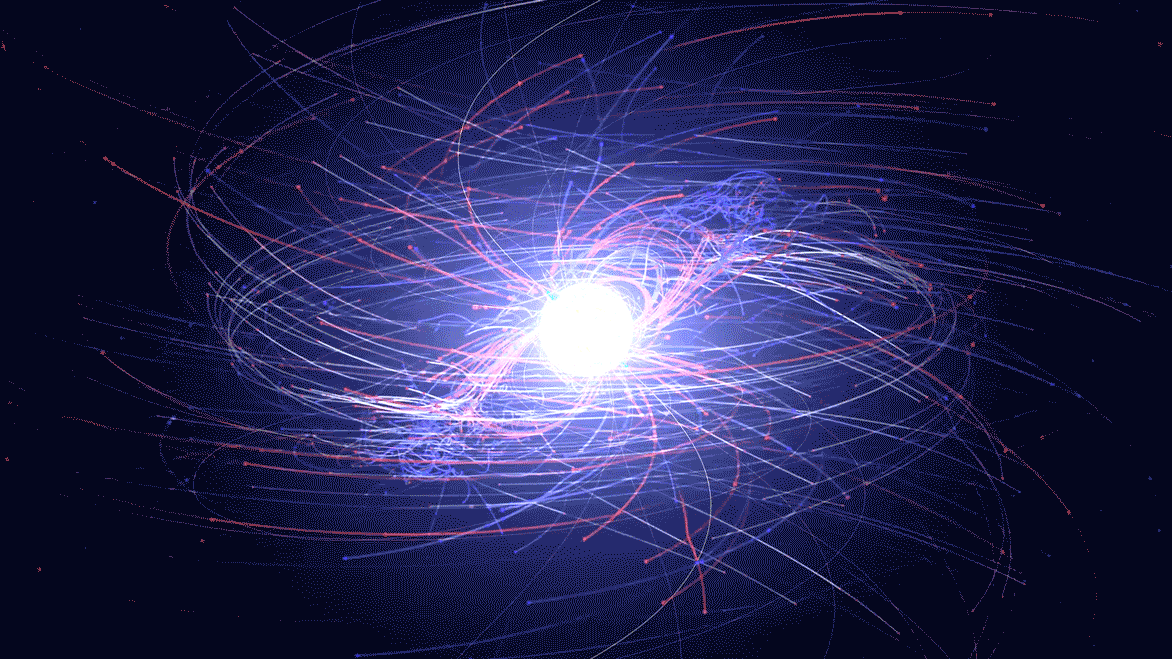
At present, the strongest direct constraints on the size of one or more extra dimensions comes from precision molecular spectroscopy, showing that the size of any such dimensions, as seen by the gravitational force alone, must be smaller than ~0.6 microns. This corresponds to an energy scale that’s around a factor of 100 higher than the scale of electroweak unification (about ~100 GeV), which puts the kibosh on the original motivation for the large extra dimension scenario: explaining why gravitation is so much weaker than the other forces we observe.
There was a lot of hope among particle physicists, in the pre-LHC era, that some type of new fundamental particle or interaction — something other than the Higgs boson, something that would take us beyond the Standard Model — would be revealed at the LHC. One of the reasons the large extra dimension scenario was appealing is that, if it were true, it would lead to the expectation that the same modifications to the theory that would lead to gravity “bleeding” into these extra dimensions would both explain the weakness of the gravitational force and also would lead to a prediction of new particles appearing at LHC energies.
With data having now dashed those hopes, as no fundamentally new particles or interactions have shown their appearance in existing LHC data, some new way of stabilizing the scale of these alleged extra dimensions must be introduced. In other words, a new theoretical contortion — some additional type of new physics beyond simply hypothesizing the existence of these extra dimensions — must also be invoked.

It poses even greater difficulty if one wants to “hide” some sort of new physics in one of these extra dimensions, such as (as was suggested by the question-asker) dark matter. Somehow, dark matter would have to exist in these extra dimensions but not overlap with our own, observable three spatial dimensions, while the gravitational effect of that dark matter would somehow “leak” into our three spatial dimensions, allowing dark matter to have the clumping effect that it does.
This is why, when physicists talk about new, beyond-the-Standard-Model ideas, one of the things they talk about is the number of new “free parameters” that must be introduced to explain things. A good idea (at least, “good” to a theoretical physicist) will have a large amount of explanatory power in exchange for a relatively small number of new free parameters that are introduced. Dark energy is a good example, as with just one new free parameter, it can explain:
- the observed (accelerated) expansion of the Universe,
- the cessation of the growth of cosmic structure, in scale, below a redshift of about z = 0.7,
- the advanced age of the Universe,
- and the observed fact of spatial flatness despite a low overall matter density.
Whereas, in the case of large extra dimensions, we have to introduce multiple new parameters just to have the scenario avoid conflicting with already-existing measurements.
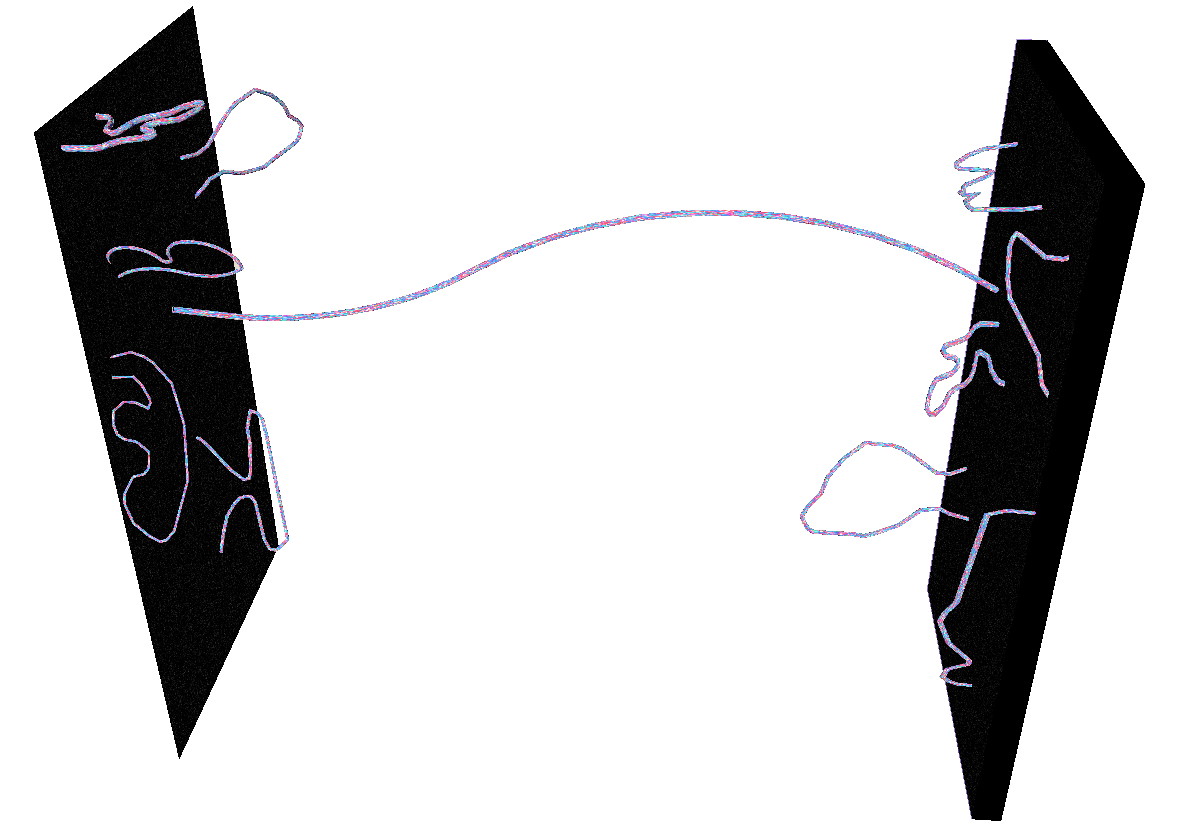
And this is the big challenge for any new theoretical idea. We don’t generally just turn a knob and say, “What if the Universe were like this?” Instead, we look to aspects of the Universe that we both can and cannot presently explain and, at the initial stage, ask two questions:
- If I make a new modification or addition to my theory, will it explain something that cannot be presently explained otherwise?
- And does that modification “screw up” any of the things I can already explain without further modifications or additions to the theory?
There are plenty of new ideas that are successful — even with just one new free parameter — but that require all sorts of theoretical contortions to survive question 2. In the case of large extra dimensions, the hope was that this modification would explain the weakness of gravity while simultaneously providing an explanation for soon-to-be-discovered particles.
With today’s (2023-era) constraints, the weakness of gravity cannot be explained by that same modification without further additions, and several issues, including:
- stabilization of the size of the extra dimensions,
- lack of proton decay,
- lack of newly discovered Kaluza-Klein particles,
- and the necessary assumption of either “hidden sectors” or other non-Standard Model states,
all plague the “large extra dimensions” scenario today. Sure, there’s no proof that a wide set of new contortions aren’t exactly what nature has in store for us, but until we find some direct evidence supporting this scenario, it will simply continue to be another “what if…” idea.
Send in your Ask Ethan questions to startswithabang at gmail dot com!