Ask Ethan: Can we see the cosmic neutrino background?
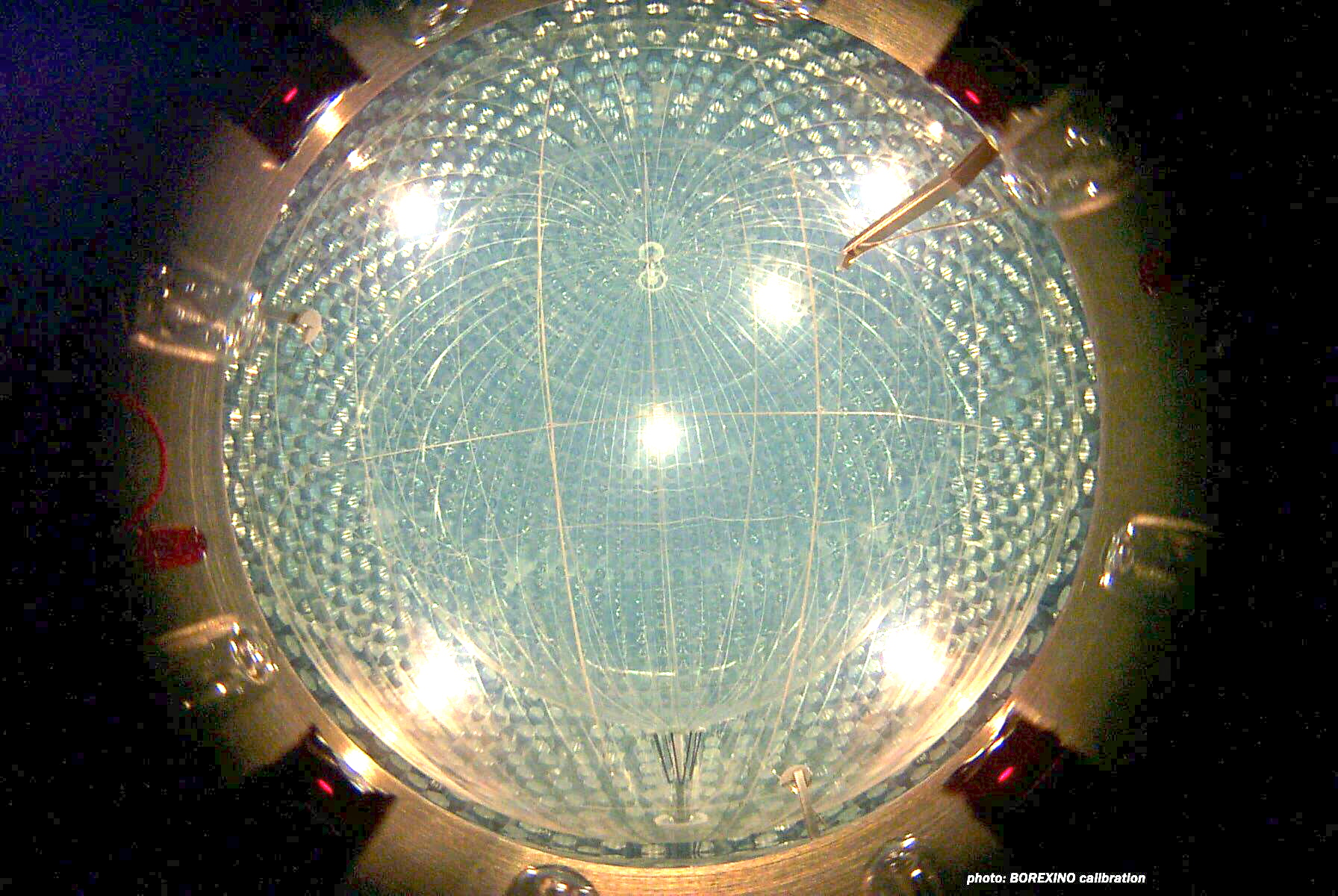
- During the earliest stages of the hot Big Bang, every species of particle and antiparticle that could possibly be produced, so long as Einstein’s E = mc² was respected, was created in vast quantities.
- As the Universe expanded and cooled, matter and antimatter annihilated away, leaving a small amount of leftover protons, neutrons, and electrons, along with two cosmic backgrounds: of photons and neutrinos.
- While the photon background was famously discovered in the 1960s, enabling us to precisely study the early stages of the hot Big Bang, the neutrino background is much more elusive. Have we detected it yet?
One of the hardest concepts to wrap our heads around is that of the hot Big Bang: the notion that our Universe began 13.8 billion years ago from an extraordinarily hot, dense, uniform, and rapidly expanding state. Initially, all known species of particles and antiparticles confirmed to exist, along with possibly others that we only speculate about at present, as there was more than enough energy to spontaneously create particle-antiparticle pairs of all types via Einstein’s famous E = mc². Since that early time, the Universe has expanded and cooled substantially, eventually giving rise to atomic nuclei, stable atoms, along with stars, galaxies, and cosmic structures on the largest scales.
But it isn’t just atoms and other structures composed of protons, neutrons, and electrons left over from that early epoch, but also cosmic backgrounds of far more numerous particles. While the relic background of photons, the cosmic microwave background (CMB), is by far the most famous leftover cosmic fossil, there should be another one composed of neutrinos and antineutrinos: the cosmic neutrino background. Reader Daniel S. Gelu wants to know about it, writing in to ask:
“My question is about if [there’s] any foreseen technology to map neutrino background radiation like CMB or BAO was already done?”
It’s an incredibly ambitious endeavor, for sure. While direct detection has not yet been achieved, we have seen the evidence for this background in a couple of different ways. Here’s the science behind the cosmic neutrino background.
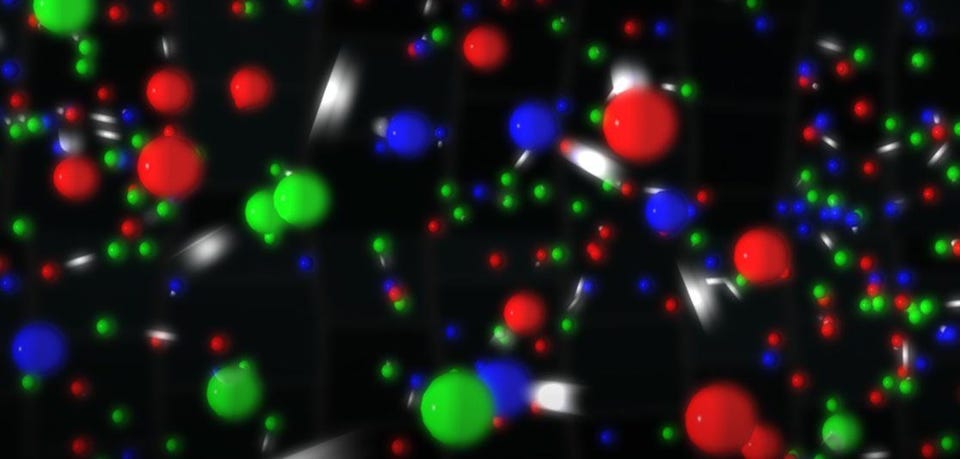
Theoretical predictions and expectations
Try and imagine, if you dare, the earliest stages of the hot Big Bang: where the energies and temperatures of the Universe were far, far greater than the energies needed to produce even the most massive of Standard Model particles. In such an environment, every particle and antiparticle that can exist does, including:
- all the quarks and antiquarks,
- all the charged leptons and antileptons,
- all of the bosons, including the photon,
- and all of the neutrinos and antineutrinos.
Although the energy scales here are still too low for quantum gravitational effects to be important, all of the known quantum forces matter: the strong, weak, and electromagnetic forces.
However, the Universe is continuously expanding and cooling. As the temperature and energy density of the Universe decrease, it becomes more difficult to produce massive particle-antiparticle pairs (limited by E = mc²), and the average time between particle interactions and collisions increases, making it easier for unstable particles to decay into their lighter, more stable counterparts. In short order — in under a second of cosmic time — most of the heavy, unstable particles have annihilated or decayed away.
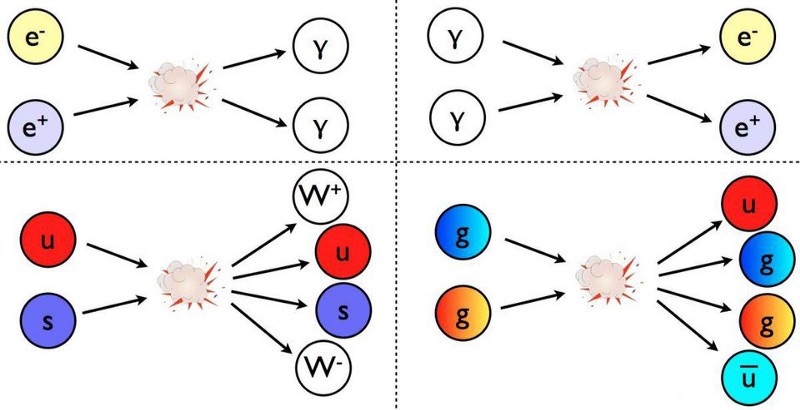
After approximately 1 second, the only particles of note remaining are:
- protons and neutrons, which have formed out of the surviving quarks,
- electrons and positrons, which are light enough that they can still be created via E = mc²,
- neutrinos and antineutrinos, which can also still easily be created via E = mc² as well as from many particle decays and annihilations,
- and photons, which also get created from particle decays and particle-antiparticle annihilations.
At this point in cosmic history, the neutrinos and antineutrinos have a very large amount of kinetic energy relative to their extremely low rest masses, so their energy distribution can be described in exactly the same fashion as the energy distribution of the photons: as following a blackbody, Maxwell-Boltzmann distribution. The only major difference is that neutrinos behave as fermions, rather than bosons (which describe photons), so they obey what’s known as Fermi-Dirac statistics, rather than Bose-Einstein statistics.
But now, something important happens. The weak interactions — the primary mechanism by which neutrinos and antineutrinos interact and are produced by — “freeze out,” which means their interactions can be ignored. Prior to this epoch, when particles and antiparticles annihilated, they were just as likely to follow weakly interacting pathways (i.e., producing neutrinos and antineutrinos) as they were to follow electromagnetically interacting pathways (i.e., producing photons). When the Universe now expands and cools just a little bit further, the electrons and positrons annihilate away, leaving only a tiny amount of electrons (to balance the electric charge from the protons) remaining, but now instead of distributing energy equally to “neutrinos and antineutrinos” on one hand and “photons” on the other hand, all of that annihilation energy now goes into photons.
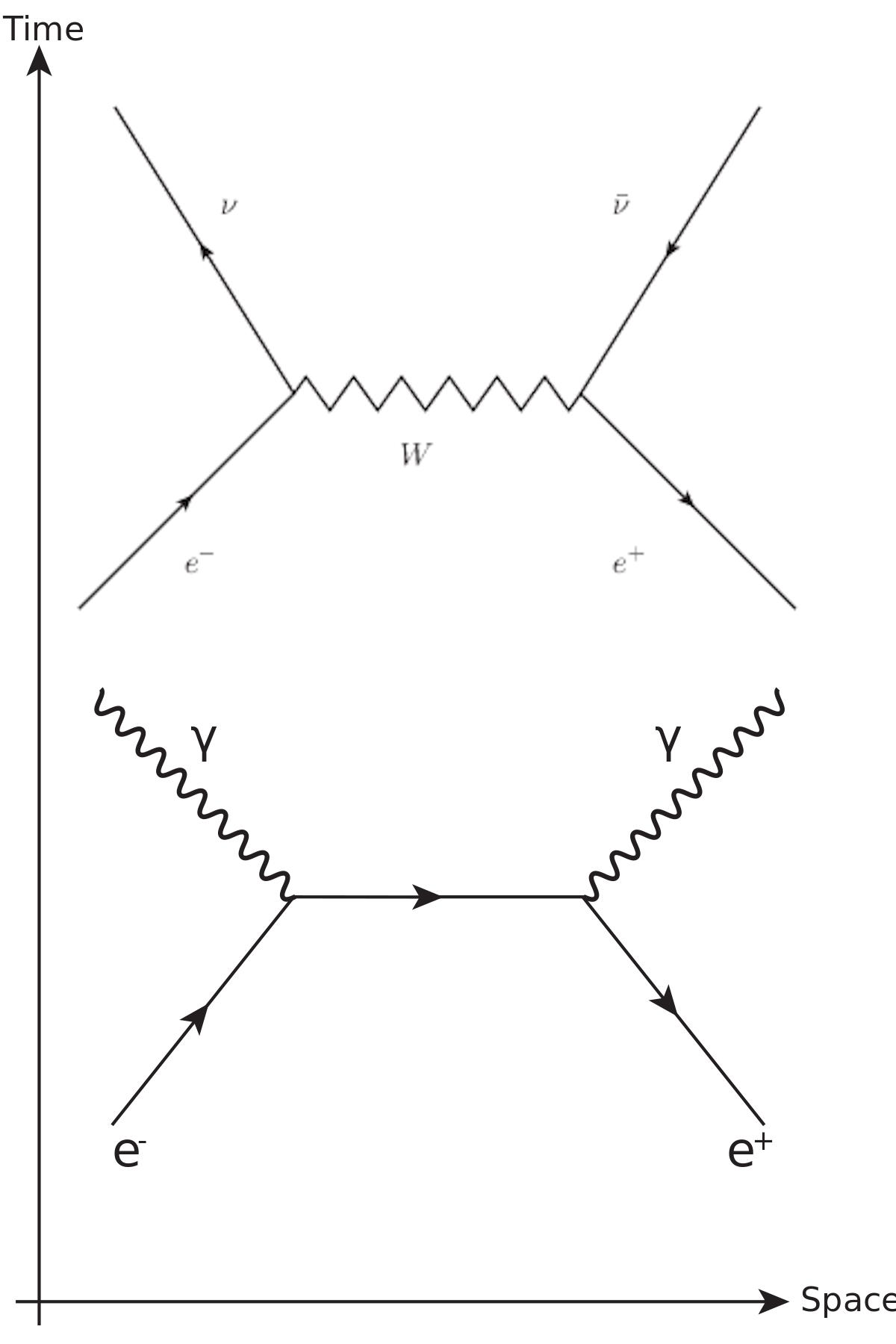
This gives a boost to the photon energy, but not to the neutrino energy. The photons, after oscillating in the leftover plasma from the Big Bang for another 380,000 years, will eventually be released as the cosmic microwave background, which we can (and do) detect today, where they’re at a relic temperature of 2.725 K. However, because the neutrinos and antineutrinos didn’t get that energy boost from the electron-positron annihilation that occurred so long ago, they should be a little less energetic. If neutrinos and antineutrinos were truly massless, the average corresponding temperature for neutrinos and antineutrinos would be a little lower: precisely (4/11)⅓ the energy of the average photon, or at 71.4% of the energy/temperature of the CMB, corresponding to more like 1.95 K.
Unlike the photons, the neutrinos and antineutrinos no longer interact/collide with either one another or with any other particle in the Universe, they only:
- experience cosmic expansion,
- contribute to the total energy density and expansion rate,
- and slow down (losing kinetic energy) as the Universe expands.
Owing to their tiny but non-zero masses, they should still exist today, eventually falling into galaxies and clusters of galaxies at late times. One of the holy grails of modern Big Bang cosmology would be to directly detect this background of cosmic neutrinos and antineutrinos, but that’s an enormous experimental challenge.
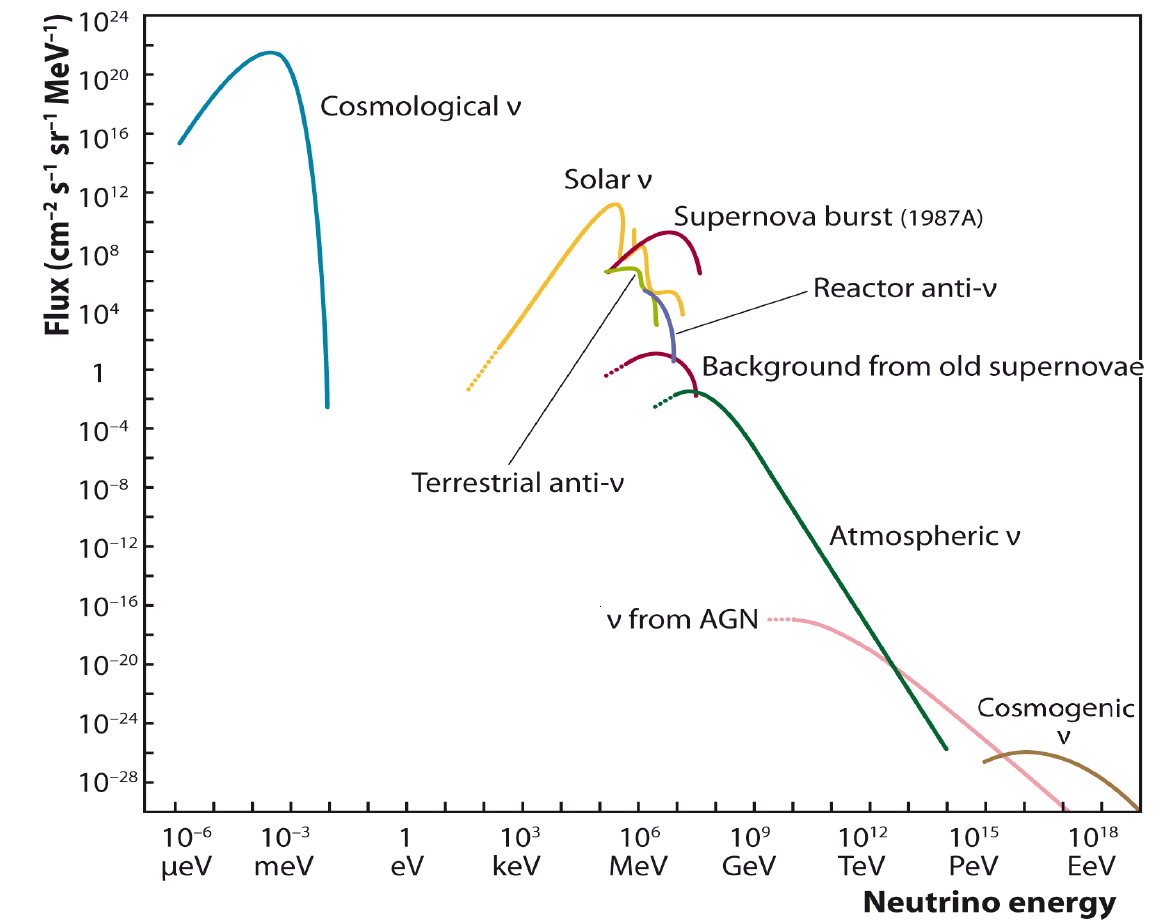
Direct detection and its near-impossibility
This cosmic neutrino background (CNB) has been theorized to exist for practically as long as the Big Bang has been around, but has never been directly detected. There are presently four observational cornerstones anchoring the Big Bang theory as our preferred theory of the early Universe:
- Hubble expansion and the redshift-distance relation,
- the observed formation and growth of large-scale structure in the Universe,
- the observation of the leftover photon glow from the Big Bang: the cosmic microwave background,
- and the abundance of the light elements, hydrogen, helium, lithium and their isotopes, created during Big Bang nucleosynthesis.
If we could detect the cosmic neutrino background, it would provide us with a fifth cornerstone for Big Bang cosmology, which would be another tremendous triumph for our understanding of the cosmos.
This is easier said than done, however. Neutrinos have an extremely tiny cross-section for interacting with other particles, and that cross-section scales with energy: higher energy neutrinos have larger interaction cross-sections with other Standard Model particles than lower energy neutrinos do. Because of that, we generally need neutrinos (and antineutrinos) to be at very high energies in order to see them. The energy typically imparted to each neutrino and antineutrino remaining from the Big Bang corresponds to only 168 micro-electron-volts (μeV) today, whereas the neutrinos we can measure have many billions of times as much energy: in the mega-electron-volt (MeV) range or higher.
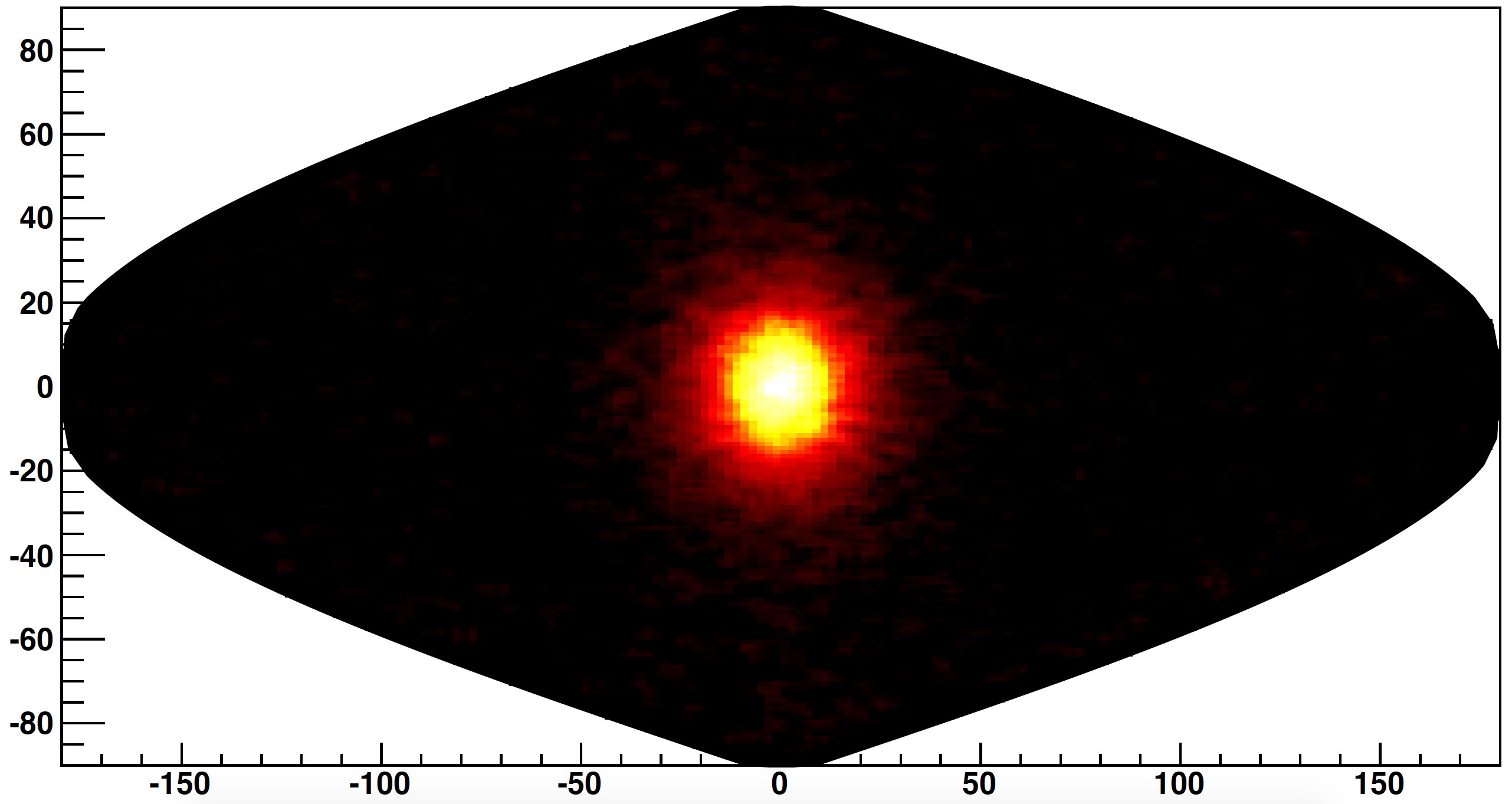
For example, above, you can see an image of “the neutrino sky” as seen by an underground neutrino observatory. That big bright spot that you see, unsurprisingly, is the Sun, which produces neutrinos (and antineutrinos) in the nuclear reactions in its core. We’ve also seen neutrinos from (high-energy) cosmic ray showers, from supernova events that have occurred within our Local Group, and (extremely rarely) from extragalactic energy sources. But these same detectors, the ones that see neutrinos with millions, billions, or trillions of electron-volts in energy, are not capable of measuring the tiny nuclear recoils that would occur from these leftover Big Bang neutrinos and antineutrinos.
In fact, there are no proposed experiments that are even theoretically capable of seeing the signals, directly, from this relic background of cosmic neutrinos unless some novel, exotic physics is at play, such as the existence of a non-Standard Model neutrino. The only way to see these neutrinos within the realm of known physics would be to build a neutrino detector and then accelerate it to relativistic speeds, which would effectively “boost” the relic Big Bang neutrinos and antineutrinos up to detectable energies: a technological implausibly scenario at present.

Indirect detection
When we detected the cosmic microwave background in the 1960s, we did it directly: we saw an all-sky signal (but not from the ground) that only varied when we looked at the plane of the Milky Way or directly at the Sun. It appeared to be “blackbody” and at the same temperature everywhere else, all throughout the day and night, with no discernible variations. Over time, as our measurements became more refined, we saw that there was a dipole moment to this signal at about the 1-part-in-800 level: evidence of our motion relative to the cosmic microwave background. And beginning in the 1990s, we detected ~1-part-in-30,000 variations, detailing the imperfections imprinted by inflation on the early Universe.
No such direct signal, even that basic, all-sky “monopole” signal, has a realistic prospect of being detected in the foreseeable future when it comes to neutrinos. But these neutrinos and antineutrinos, which existed with specifically predicted properties (including number density, energy-per-particle, and the shape of their energy distribution spectrum) at even extremely early times during the hot Big Bang, could still have their signatures revealed indirectly: through the neutrino imprints on signals that are directly observable. Imprints from the cosmic neutrino background should show up in:
- their effects on the CMB, or cosmic microwave background,
- and through their imprints on baryon acoustic oscillations, a feature found in the large-scale structure of the Universe.
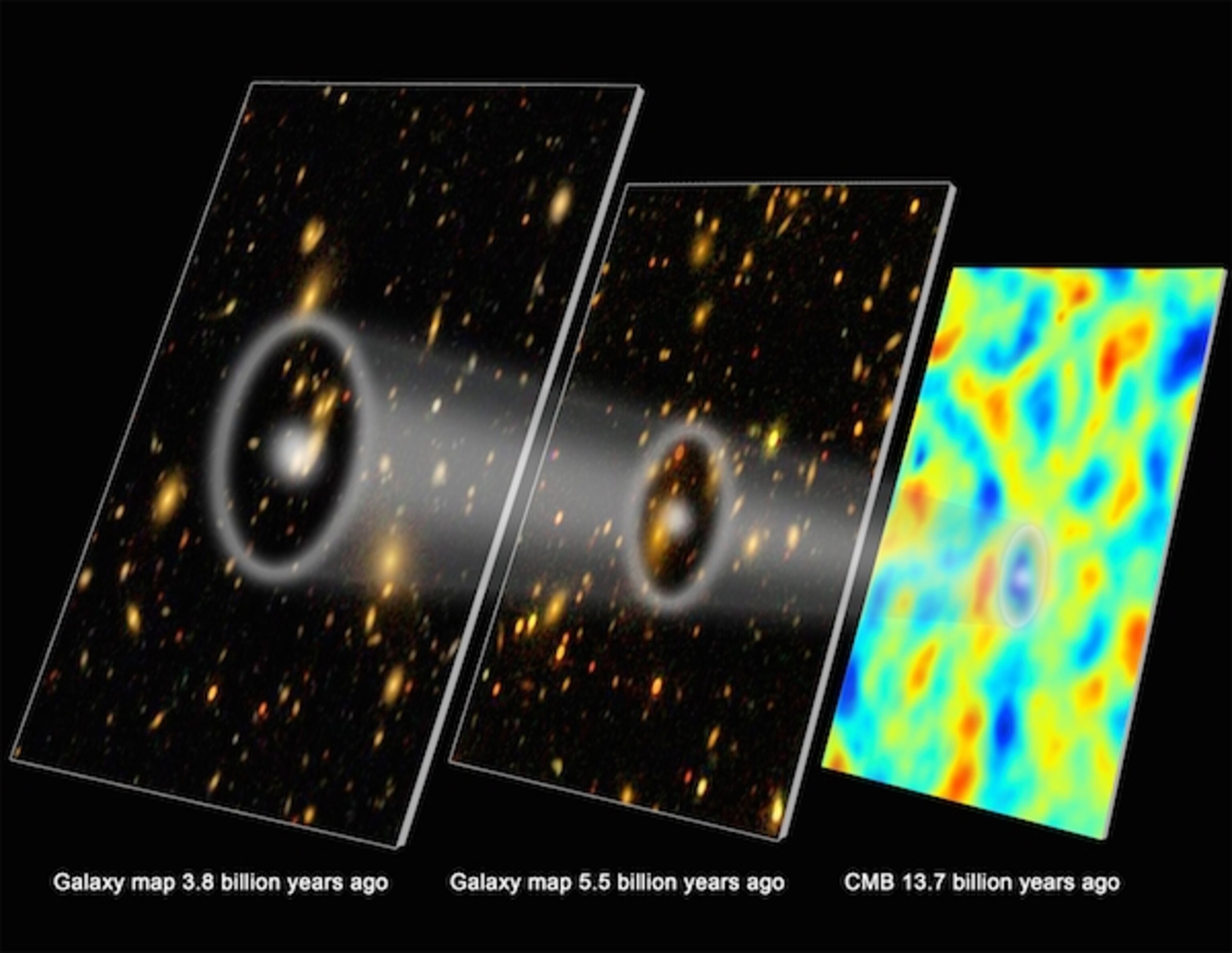
The way they do this is simple to envision: early on, neutrinos behave like a form of radiation, as they move at speeds indistinguishably close to the speed of light. Unlike photons, however, they don’t collide or interact with matter; they just pass through it. Therefore, where you start to form gravitationally bound structures — i.e., when gravitational imperfections begin to grow — the neutrinos stream out of those structures, smoothing-out the seeds of what will eventually form star clusters, galaxies, groups-and-clusters of galaxies, and even larger-scale structures than that.
If there were no radiation, those initially overdense clumps of matter would grow unencumbered, driven solely by gravitational collapse. If there were only photons, then the denser a structure grew to be, the greater the amount that photons would “push back” against that growth, causing a bouncing effect and leading to peaks-and-valleys in the magnitude of structure on different cosmic scales. But if you now add neutrinos into the mix, they shift that pattern of peaks-and-valleys out to (slightly) larger cosmic scales. In terms of observables, that translates into what we call a “phase shift” in the fluctuation pattern seen in the cosmic microwave background, dependent on the number of neutrino species that exist (which should be exactly 3: electron, muon, and tau) and the temperature/energy of those neutrinos (which, again, should be precisely (4/11)⅓ of the photon temperature/energy) at that critical, early time.
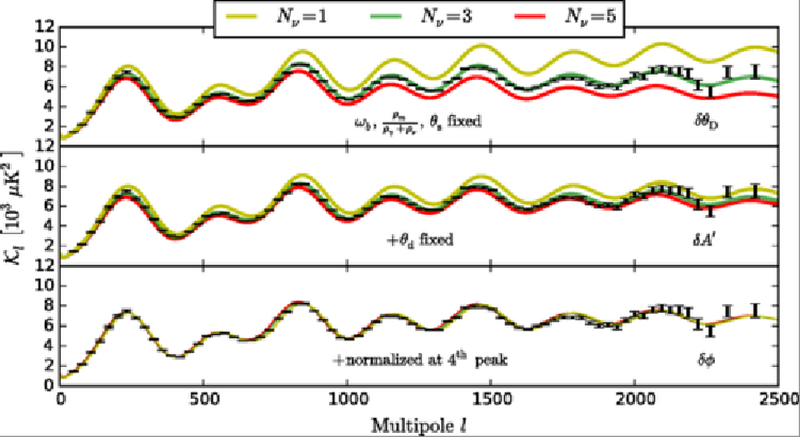
In 2015, using state-of-the-art data from the ESA’s Planck satellite, a quartet of scientists published the first detection of the cosmic neutrino background’s imprint on the relic light from the Big Bang: the CMB. The data were consistent with there being three and only three species of light neutrino, consistent with the electron, muon, and tau species we’ve directly detected through particle physics experiments. By specifically examining the polarization data from the Planck satellite, as first reported at the January 2016 meeting of the American Astronomical Society, the team was also able to determine the average energy inherent to each neutrino present within the cosmic neutrino background: 169 μeV, with an uncertainty of only ±2 μeV, in precise agreement with theoretical predictions of 168 μeV. It was an astounding and monumental accomplishment, indirectly supporting the existence of the cosmic neutrino background.
But anything that shows up in the cosmic microwave background should have downstream effects as well, because those are the very seeds that will grow into the large-scale structure that fills our observable Universe today. The imprint, just as is the case with the CMB, should be subtle, but should create a detectable signature in how galaxies correlate with one another, population-wise, across the cosmic distances. If you put your finger down on any one galaxy in the Universe, there’s a specific probability of finding another galaxy a certain distance away from it, and the presence and properties of neutrinos can affect that distance scale as well. That scale, moreover, will evolve with cosmic time: as the Universe expands, that scale expands as well.

In 2019, just a few years after the CMB signal indicating the presence of the cosmic neutrino background was detected, a team of scientists led by Daniel Baumann, working with data from the Sloan Digital Sky Survey, revealed the offset of the matter-radiation interaction signal caused by neutrinos, and again found it was consistent with the predictions of standard Big Bang cosmology. It also placed very tight constraints — perhaps the first meaningful constraints — on the possibility that neutrinos and dark matter would interact. Because the acoustic scale (the scale of peaks-and-valleys) that was seen showed no bias in either direction, this ruled out a variety of models that do have strong neutrino-dark matter interactions.
We can be extremely confident that the cosmic neutrino background does exist, as we’ve detected evidence for its existence by their imprints in both the cosmic microwave background and in the way that galaxies cluster within the large-scale structure of the Universe. Even though we haven’t detected these cosmic neutrinos directly, these two pieces of indirect evidence, which are good enough to rule out, in each case, the possibility that there isn’t a cosmic background of neutrinos at all. (Although there is still wiggle-room for non-standard neutrinos to be viable.)
With the first signals that the cosmic neutrino background is real, and with increasingly precise CMB observations and better large-scale structure surveys on the horizon — including ESA’s Euclind, NASA’s Nancy Roman space telescope, and the NSF’s Vera Rubin observatory — the Big Bang may yet get a fifth cornerstone supporting its validity. Direct detection of this background, however, is still very far off. Perhaps some clever, future scientist is reading this piece right now, and they’ll be the ones who’ll figure out just how to best detect this early, elusive signal, left over from just ~1 second after the Big Bang!
Send in your Ask Ethan questions to startswithabang at gmail dot com!