The CMB: The most important discovery in cosmic history

- First theorized to exist back in the 1940s, the leftover glow from the Big Bang — also known as the cosmic microwave background, or CMB — was confirmed to exist back in the mid-1960s.
- Although many have questioned its interpretation and ultimate origin, its detailed properties not only confirm its emergence from a hot Big Bang, but provide many other cosmic insights.
- Yet still, even today, one huge cosmic mystery looms large: the Hubble tension. Here’s what we know about the CMB, plus what still remains to be discovered about our Universe.
There have been a few momentous discoveries throughout the history of science that have literally revolutionized our understanding of the Universe. The relationship between electricity and magnetism, the deflection of starlight by the gravity of the Sun, the fact that matter and energy are quantized into individual packets and particles, and the fact that the Universe is expanding were all examples of extraordinary discoveries that fundamentally changed how we viewed the Universe. And yet, there’s one discovery that’s arguably even more important than all of those in our quest to understand the nature of reality and the origin and history of our Universe: the CMB, or the cosmic microwave background.
Discovered quite by accident in the mid-1960s, its discovery not only validated the Big Bang while refuting all then-viable competitors, but ongoing, more refined measurements have taught us an enormous suite of cosmic lessons that have continued to push the frontiers of our understanding for 60+ years, and counting. While there are many conundrums that loom large in cosmology today — from the Hubble tension to hints of evolving (or weakening) dark energy — the CMB remains, in many ways, our best probe of the early Universe and how it evolved into all the stars, galaxies, planets, and more that we see today. Here’s how the CMB brought about our modern understanding of the Universe.

The story begins back in the 1920s: when three great advances, one theoretical and two observational, all got put together for the first time.
- Vesto Slipher’s observations that the spiral and elliptical nebulae, seen ubiquitously in the sky, exhibited spectral line shifts that indicated they were not just moving rapidly, but that they were largely receding away from us.
- Edwin Hubble’s observations of individual (Cepheid variable) stars within those nebulae, allowing us to determine a distance to them. When combined with Slipher’s data, they showed that the farther away a distant spiral/elliptical object was from us, the faster it seemed to be receding.
- And Alexander Friedmann’s theoretical work, showing that a Universe filled uniformly with any-and-all forms of matter, radiation, or other species of energy cannot remain static and stable, but must either expand or contract.
The fact that we lived in a Universe that was, on the largest scales, seen to be (roughly) uniformly filled with matter in all directions and all locations, taught us that Einstein’s original vision of our Universe — that it was static and eternal — couldn’t be right. Instead, as Slipher’s and Hubble’s observations showed, the Universe was evolving, and the specific way it was evolving is that it’s expanding: the farther away an object is from us, the faster it appears to be moving away from us.

Although this provided the seed for what would become the theoretical framework of the Big Bang, there were many other possible explanations for an expanding Universe. The Universe could have been rotating, oscillating, creating new matter in the space between galaxies, having light get “tired” (or lose energy) as it travels through space, along with many others. But it was also possible that the Universe — because it was expanding today — was smaller, denser, and (because of how temperature works) hotter in the past. This extrapolation was considered by many in the 1920s and 1930s, but the person who brought it to the forefront was George Gamow, himself a former student of Friedmann’s, in the 1940s.
Gamow, along with others, realized that if the Universe were smaller, denser, and hotter in the past, then there would be three observable consequences in addition to the already-observed expanding Universe.
- Structure formation would be hierarchical, with large galaxies only appearing at late times, smaller, less evolved galaxies appearing earlier on, and beyond a certain point, no stars or galaxies at all.
- At very early times, the Universe would have been hot and dense enough to forge atomic nuclei through nuclear fusion, predicting that elements other than hydrogen would have existed even before any stars were formed.
- And, at some point, the Universe “cooled” sufficiently to stably form neutral atoms, leaving a relic bath of radiation permeating the Universe.
Because the Universe has expanded and cooled in all the time since those early stages, that leftover radiation — originally called the primeval fireball (and later known as the cosmic microwave background, or CMB) — should only be a few degrees above absolute zero by today.

Gamow’s ideas didn’t dominate the field in the 1940s and 1950s; they were considered as a possibility, but the evidence just wasn’t there. Then, in the 1960s, a team at Princeton — led by Bob Dicke but including Jim Peebles, Peter Roll, and David Wilkinson — decided to look for that leftover background of radiation. They built and intended to fly a device, a Dicke radiometer, that would be able to detect that microwave radiation. Unfortunately, they’d never get the chance.
That’s because, just 30 miles away in Holmdel, NJ, two researchers at the Bell Labs, Arno Penzias and Robert Wilson, had a new antenna designed to make cutting-edge radar measurements: the Holmdel Horn Antenna. But something seemed to be wrong: whenever they observed the sky, in any direction, day or night, they kept seeing a noisy “hum” that they couldn’t eliminate. It didn’t exist when pointed at the ground, and it varied when pointed at the Sun or the galactic plane, but otherwise, it was just a constant source of signal.
After several months, a visitor pointed them to the work of the Princeton group, and it was swiftly realized what they were seeing: in all directions, a bath of uniform temperature in the sky. They had just discovered the Big Bang’s leftover glow: the CMB.

But was it really a sign of our cosmic origins? Many put forth alternative explanations, attempting to avoid this unpalatable conclusion.
- Perhaps it was just the existence of all the starlight throughout the Universe, summed together and reflected off of other matter: dust and gas clouds.
- Perhaps it was a foreground: from within the Solar System or within the Milky Way galaxy, that was emulating the expected properties of the CMB.
- Perhaps it was a relic from the earliest stars formed in the Universe: from so-called Population III stars, whose light has been redshifted by the expanding Universe in all the time since.
- Or perhaps there was another (radical) solution that was admissible: reliant on starlight, heating, and other forms of radiating matter.
One way to tell would be to not just measure the existence of the CMB, but its spectral properties: how its energy varied, both across the sky (from place-to-place) and in terms of its intensity as a function of frequency.
The intensity vs. frequency question is perhaps the most stringent test: the CMB is predicted to not just have the properties of a blackbody — the spectrum of a perfectly black object heated up to (and radiating at) a specific temperature — but to have the properties of the most perfect blackbody ever created in the Universe. You might think of a star as a blackbody, but the truth is that for any star you consider, including our own Sun, it behaves only as an approximate blackbody, and not a very good one at that when you examine it in detail.

The Sun, like all stars, isn’t a single, solid object heated up to a uniform temperature. Instead, it has:
- starspots, or regions across its surface where its temperature is lower (and so it appears less luminous) than others,
- absorption features, which are due to light-absorbing elements (atoms and ions) found in its outer layers,
- and a temperature gradient from the outer layers of its photosphere to innermore ones, all of which emit light that makes its way out to the broader Universe.
Each individual star has its own unique properties: its own average temperature, its own temperature gradient, its own abundance of various elements to absorb light, its own temperature variations throughout time, as well as displaying flares, space weather, and other variable activity.
When you observe the spectrum of any individual star in detail, you find that it cannot be well-approximated by a single blackbody, but rather is better-approximated as the sum of a series of blackbodies: something that gives a very different spectral signature than a single blackbody. One of the goals for scientists working with the CMB was to measure its intensity as a function of frequency/wavelength very, very well, determining whether it’s a perfect blackbody (as predicted by the Big Bang) or whether it’s more consistent with any other shape (demanded by all alternatives). As the data collected throughout the late 1960s, the 1970s, 1980s, and (especially) with the COBE satellite in the 1990s all showed, it is indeed the most perfect blackbody in the entire Universe.

But then we have to ask ourselves: is it the exact same temperature everywhere? The first hints that the answer was “no,” came about 10-15 years after its initial discovery: when our measuring devices became sensitive enough to measure about 1-part-in-800 variations, or ~millikelvin variations in a radiation bath that was only about 3 K hotter than absolute zero. Remarkably, the temperature showed a peak, or “hot spot,” in one direction, and then a trough, or “cold spot” that was equal-and-opposite to the “hot spot” in both magnitude and direction, exactly.
Was that evidence that the Universe was doing something unexpected?
Not at all! That was evidence that we — the observers of the CMB — are in motion with respect to the Hubble flow, or the expansion of the Universe. In particular, that temperature variation we see, of ±3.346 mK, corresponds to a motion of around 370 km/s: about 0.1% the speed of light, consistent with the peculiar motions of most cosmic objects (stars, galaxies, etc). We’ve refined these measurements exquisitely since the first hint of “the cosmic dipole” was measured back in the 1970s, and everything we see is consistent with this interpretation. Only by subtracting out:
- the average temperature of the Universe, or 2.7255 K,
- and then the CMB dipole, with temperature variations of ±3.346 mK corresponding to our motion of ~370 km/s through the Universe,
can we finally start to see the primordial fluctuations, or imperfections, imprinted in the CMB.
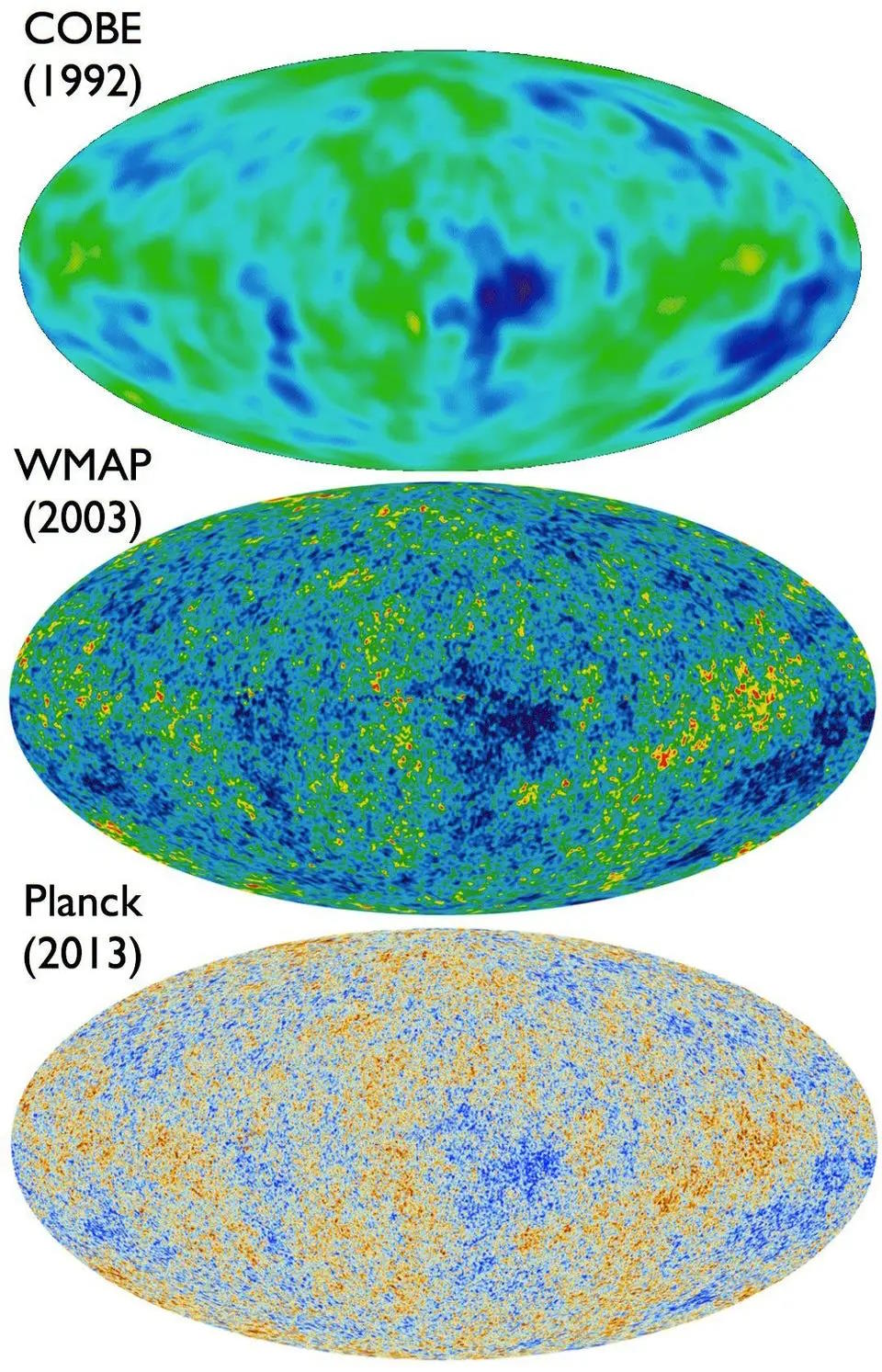
First seen by COBE, then BOOMERanG and MAXIMA, then WMAP, and then Planck, these fluctuations obey a specific set of patterns, or “wiggles,” that show up as temperature differences from the “average” temperature of the CMB (with the dipole motion subtracted out).
These temperature fluctuations, or anisotropies (differences in different directions), are tiny: showing up at only the 1-part-in-30,000 level, on average. However, you’ll notice, if you look at the fluctuations in detail, that they show:
- a flat distribution, or that 1-in-30,000 departure from the average temperature, on large cosmic scales,
- then increase to a peak almost two-and-a-half times as large at smaller (~1°) angular scales,
- then decrease again, with periodic “peaks” and “troughs” showing up as wiggles in the graph,
- until, at scales below about 0.1°, the temperature fluctuations decrease to less than their original values.
This information tells us two important things about the Universe. First, the ratios of the heights and locations of these peaks and valleys, or wiggles, show us the relative ratios of normal matter, dark matter, radiation, dark energy, and spatial curvature in the Universe. There isn’t one unique solution, but rather an array of multiple (what we call “degenerate”) solutions: possible solutions that require some other measurements to help us constrain them.
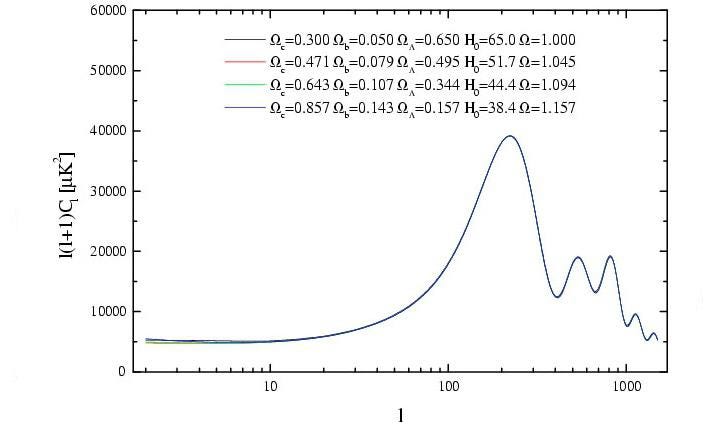
But the second thing it tells us is arguably even more profound. By understanding the physics of the early Universe — how ionized particles and photons interact, as well as how dark matter does and doesn’t interact — and combining it with what we know the Universe is made of, we can extract all sorts of other cosmological parameters. These include:
- the age of the Universe,
- the physical scale of acoustic oscillations (the “wiggle” features in the CMB) in the Universe,
- the size of the Universe back when it was just 380,000 years old (when the CMB was emitted),
- and, based on all of that, the expansion rate as well.
The CMB is an exquisite source of information for all of these factors, and even despite the degeneracies mentioned above, an incredibly precise probe that pins down what these parameters are allowed to be when all taken together.
There’s lots of independent evidence supporting the general consensus picture given to us by the CMB:
- a Universe that’s 13.8 billion years old,
- a Universe whose structural seeds were provided by cosmic inflation,
- a Universe that’s ~68% dark energy, ~27% dark matter, ~4.9% normal matter, and ~0.1% neutrinos and radiation,
- a Universe with no hint of spatial curvature that departs from zero,
and one that’s controversial: a Universe that’s expanding at a rate of 67 km/s/Mpc.
The reason that’s controversial? Because that expansion rate uses a method of measurement which starts far away and then comes forward, giving us a value of 67 km/s/Mpc. On the other hand, methods that start nearby and then go farther out give a different, inconsistent value: 74 km/s/Mpc.

This puzzle is what we currently call the Hubble tension, and it has only intensified with new data from a variety of cosmic sources. It’s possible, albeit unlikely, that our interpretation of the CMB has slightly fooled us — perhaps due to the same kind of “incorrect” foreground subtraction that led to earlier “discoveries” that since went away (early reionization, a running spectral index, B-modes attributed to inflation, etc.) — and that we’re actually inhabiting a Universe:
- that’s got a little more dark energy,
- that has a little less dark and normal matter,
- that’s a tiny bit younger,
- and that’s expanding a tiny bit faster,
than current CMB results indicate.
But it’s also possible that this tension, and the continued strength of the full suite of CMB observations, is actually a hint of either new physics or astrophysics. Perhaps there was something that happened early on in cosmic history that altered the scale of the temperature fluctuations we see, and we’re not accounting for it. Perhaps there was an exotic species of energy, still undiscovered, that decayed early on into some other form of matter or energy. Perhaps there are flaws with how we’re interpreting all sorts of components of the full suite of cosmic data. Or, quite possibly, it’s possible that something novel, expected, and revolutionary is occurring within our Universe, and these are where the first hints are showing up.
However the Hubble tension shakes out, the CMB will remain one of the most powerful and extraordinary pieces of evidence that must be reconciled with whatever cosmic picture we come up with. For, in the end, we can only draw conclusions about how the Universe actually is from the information it tells us about itself.