Ask Ethan: Is it all-or-nothing for the expanding Universe?
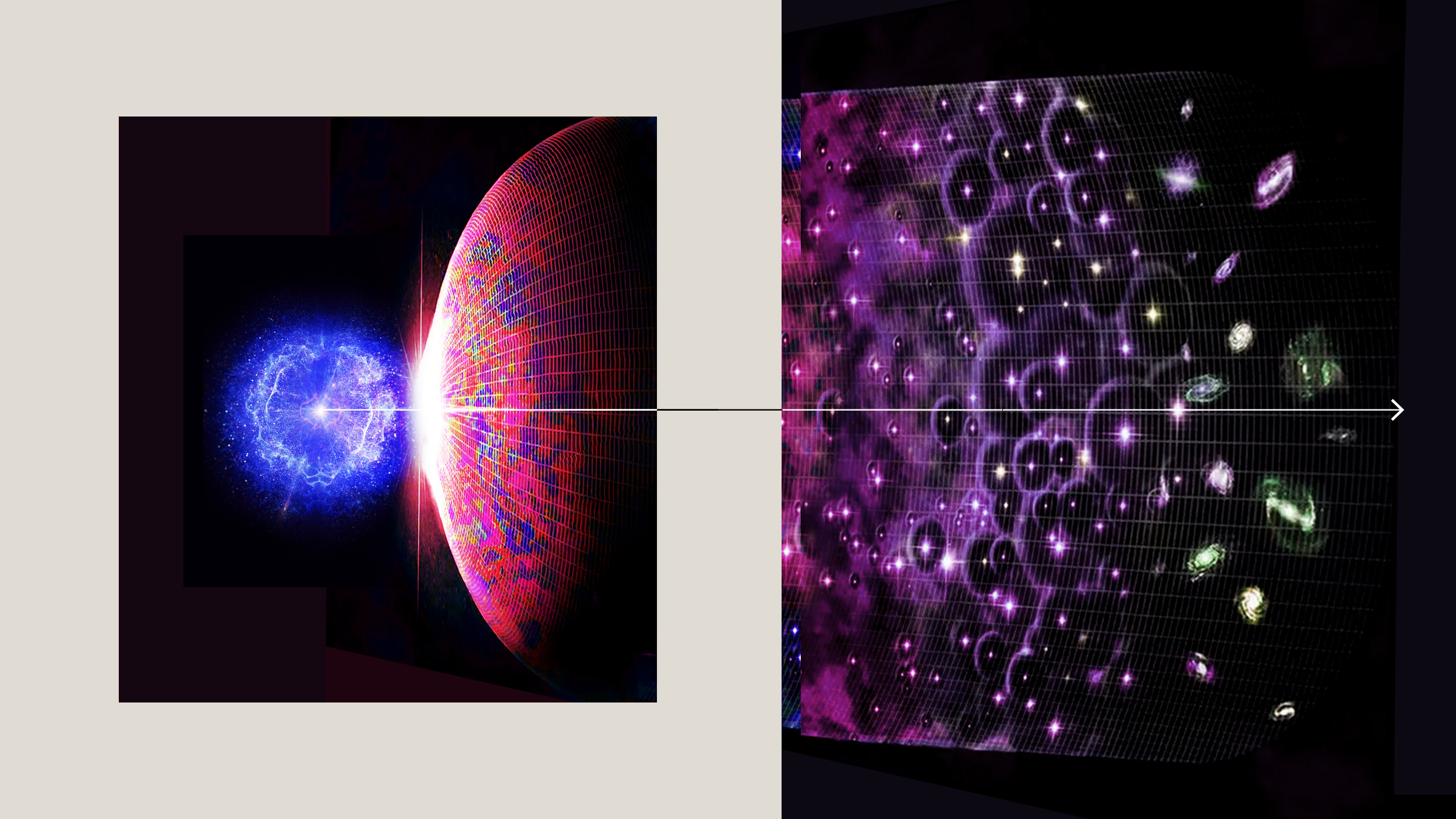
- Ever since the 1920s, nearly a full century ago, humanity uncovered that most galaxies, on average, are mutually receding from one another, marking the birth of the discovery of the expanding Universe.
- However, that expansion only takes place in the space between bound objects, like galaxies or galaxy clusters, and doesn’t apply to regions that have already collapsed, like supermassive black holes.
- Some have proposed theories that the Universe is cyclical: expanding-and-collapsing periodically, while others wonder about eventual recollapse on unobservably large scales. Here’s what we know.
One of the most remarkable revelations in our understanding of the cosmos is the fact that the Universe is expanding. Distant galaxies, on average, all appear to recede from us, with faster and faster recession speeds for galaxies that exist at greater distances. While individual objects and systems may be gravitationally bound together — stars and planets, galaxies, plus galaxy groups and clusters — the space between these structures is not only expanding today, but has been expanding for all 13.8 billion years of cosmic history, and will continue to expand indefinitely far into the future as well.
But is the expanding Universe truly an all-or-nothing proposition? Are there exceptions to the expanding Universe, and is “collapse” a scenario that’s been ruled out entirely? That’s the question plaguing the mind of Patreon supporter Brent Minder, who wants to know:
“Does the universe’s current state have to be all or nothing in terms of expanding or collapsing? Is there a theory that the universe is in a mid-state of collapse and expanse? That is: what if the universe has been expanding and collapsing forever and the black holes that “formed too soon to exist” are remnants from a previous expanse? The farthest parts of the universe could be too far away to collapse back, but could the ‘centermost’ areas collapse back upon themselves — or are they moving away at crazy fast speeds as well?”
There are a lot of questions here, so let’s start not with what we observe, but with a theoretical view to how, and whether and when, the Universe does (or doesn’t) expand.

Back when Einstein was first developing general relativity, he recognized that there was a tremendous problem with this new theory of gravity. Everywhere we looked, we saw points of light throughout the sky: in all directions, distributed relatively equally, at all distances, as far as we could see. Despite the fact that there are very dense clumps of matter — things like individual stars, for example — and then vast swaths of interstellar space that are largely devoid of matter, on the largest scales we could observed, things looked both isotropic (the same in all directions) and homogeneous (the same in all locations).
And yet, if you solve the Einstein field equations for a Universe that is both isotropic and homogeneous, you find something shocking: your Universe would be unstable, and would collapse directly into a singularity, such as a black hole. It’s not just that the matter would contract within the space of the Universe, but space itself would contract, falling in on itself, and dragging all the matter in that space in along with it.
Einstein noticed this pathology, and since the Universe clearly hasn’t already collapsed and doesn’t appear to be collapsing right now, something was wrong with this picture. Since this couldn’t be the case, Einstein felt compelled to resort to drastic measures.
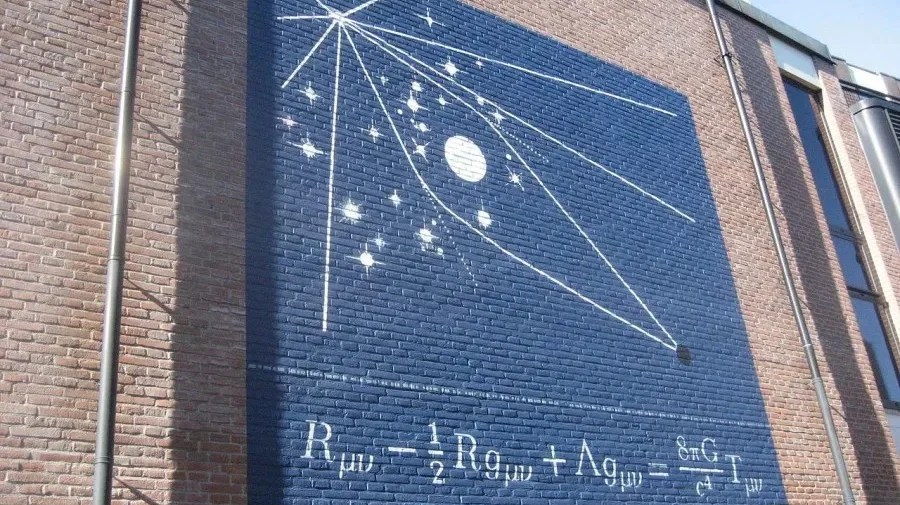
“What holds the Universe up against collapse?” he must have wondered. All matter and radiation that exists is gravitationally attractive, overall, and would contribute to the Universe falling in on itself, leading to a contracting-and-collapsing scenario. However, Einstein recognized that if he added a new form of energy that was neither matter nor radiation — a form of energy that was intrinsic to space itself: a cosmological constant — it could counteract that contracting/collapsing impetus through an outward type of pressure. If the cosmological constant were large enough in magnitude, at least, large enough compared to the matter-and-radiation densities, the Universe might not contract, but instead could remain stable.
Of course, balancing matter-and-radiation with a cosmological constant is a tricky business. If your Universe expands just a little bit, the matter-and-radiation density drops while the cosmological constant density stays constant, and so expansion swiftly wins by greater and greater amounts. If your Universe contracts just a little bit, the matter-and-radiation density increases while the cosmological constant density stays constant, so contraction/collapse wins by greater and greater amounts. As was swiftly shown, the only way to have a Universe with (roughly) equal amounts of matter on large scales that lives for a long time is to have a Universe that:
- begins expanding,
- with gravity from matter counteracting and slowing the expansion over time,
- and where the matter-and-energy density nearly perfectly matches that initial expansion rate.
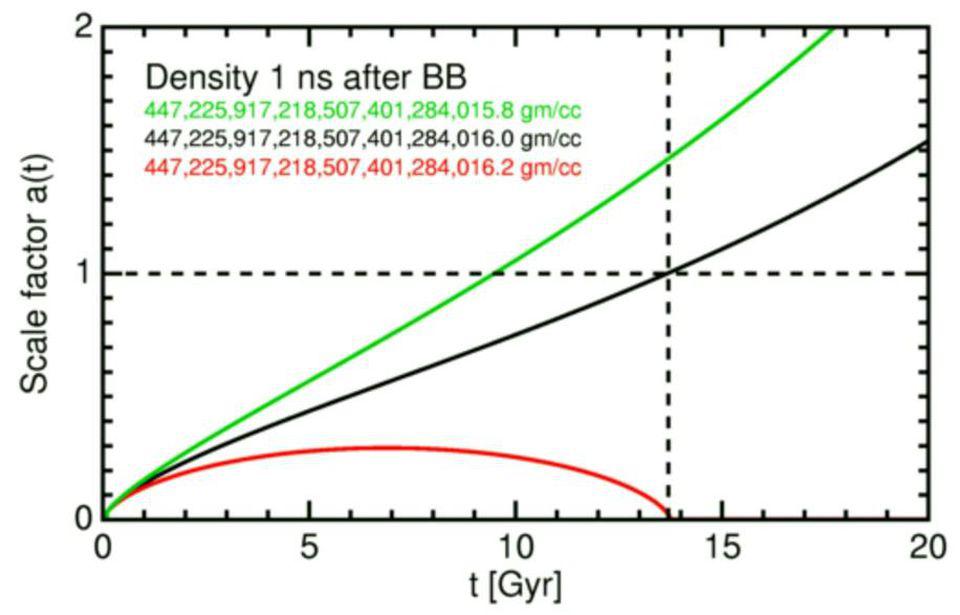
It was these theoretical concerns that first led to the notion of the expanding Universe and the idea of what we now call the hot Big Bang, as scientists began working out solutions to Einstein’s equations under various conditions just months after Einstein first put forth general relativity in 1915. Years before Edwin Hubble’s critical observations about the extragalactic nature of the spirals and ellipticals in the night sky, the seeds of what would become the modern science of cosmology had already begun to germinate.
Of course, our Universe isn’t exactly perfectly uniform at all, but only approximately uniform on the largest scales: if you imagine smearing out the individual clumps of matter — like planets, stars, and galaxies — over enormous cosmic distances. In reality, there are tiny imperfections that the Universe is seeded with:
- regions of slightly greater-than-average density,
- regions of approximately average density,
- and regions of below-average density,
all adjacent to, and overlapping with, one another. These “seed imperfections” exist on all scales as far as we can tell and measure, from the largest cosmic ones down to scales of a tiny fraction of a square degree in the sky. Over time, under the influence of gravity, the denser regions “steal” matter, preferentially, away from the surrounding less dense regions, and the result, over long enough periods of time, is a great cosmic web.

You might then think to ask, “Okay, if the Universe is expanding on average, but I have these overdense regions (that gravitationally grow) and these underdense regions (that give up their matter) within it, what determines whether things continue to expand, or whether they gravitationally collapse?”
If you thought about this, then you’re already on the right track. As you might imagine, a region of below-average density will get less and less dense over time, and because it has less matter in it, it has less “stuff” to gravitationally fight the expansion. As a result, these regions grow larger and larger, expand faster than average, and tend to grow into cosmic voids: the spaces between galaxies, galaxy groups, and galaxy clusters.
On the other hand, the above-average-density regions will expand more slowly than the cosmic average, and over time, will draw greater and greater amounts of matter into them. At some critical point, a threshold will be reached: where enough matter has fallen into an overdense region that space no longer expands within this region. Instead, it becomes a region where space is gravitationally bound, and just like a black hole, this gravitational overdensity, even if it doesn’t create an event horizon or a singularity, and even if it rotates, will cause the space around it to “flow” in toward the central region.

That’s what it takes to overcome cosmic expansion: to change the energy density in a given region of space so that there’s enough “binding” stuff — like normal matter, dark matter, neutrinos, black holes, and even radiation — to overcome both the impulse of the initial expansion and also the ongoing outward push from dark energy.
Now, the next key question, at least from my point of view, is to get quantitative about things, and to ask, “Okay, if that’s what needs to occur, then how much of an overdensity do you need in order to trigger some sort of binding, and how much more dense than the cosmic average do you need to be to therefore overcome the expansion?”
It turns out it’s not that much greater than average: a density in a region of space that’s about 68% greater in density than an average region is enough to do it. Below 68%, and your overdense region will only grow slowly: what we call linear growth, where the size of the overdensity increases in proportion to the ratio of the matter density of the Universe vs. the radiation density of the Universe. Even in an expanding Universe, this ratio always increases, as:
- matter dilutes proportionally to volume, so as the Universe grows by a factor of r, the matter density drops proportional to 1/r³,
- but radiation dilutes proportionally to volume plus the stretching of the wavelength of that radiation, so as the Universe grows by a factor of r, the radiation density drops proportional to 1/r⁴.

Thus, as the Universe expands, the matter overdensities grow. And once they reach that critical threshold of about ~68% greater than the average density, they start to grow much more quickly; they enter what cosmologists call the regime of nonlinear growth. Once they cross that threshold, the density now increases much more rapidly, and in very short order, the expansion of space in that region will cease. Now, in a very physically real sense, this volume of the Universe that contains all of this matter begins contracting, as it’s become gravitationally bound, and all sorts of structures — stars, galaxies, galaxy groups and clusters, etc. — begin to form inside of it, dependent solely on the scale, mass, and size of this region.
In fact, we can look to our Universe itself, at:
- the density fluctuations present in the cosmic microwave background (CMB),
- the features present in galaxy clustering statistics all throughout cosmic history,
- and the spectrum of seed fluctuations predicted by cosmological inflation,
and find that it all tells the same, self-consistent story. At the start of the hot Big Bang, inflation seeded the Universe with fluctuations that were roughly the same magnitude on all scales: about 1-part-in-30,000, on average, and those fluctuations then evolved according to both the matter and radiation in the Universe. By the time we “see” the CMB, 380,000 years have passed, and those fluctuations have grown in magnitude most severely on one specific scale, corresponding to about angular scales of 1 degree in the sky.

Even though only 380,000 years have passed from the start of the hot Big Bang to the emission of the CMB, the average fluctuation on a scale of 1 degree is now six-to-seven times larger than it was initially: about 1-part-in-5000. As the radiation density continues to drop faster than the matter density, by the time the Universe is only a few tens of millions of years old, the most severely overdense regions — the 3-sigma, 4-sigma, and 5-sigma outliers in terms of density — have already crossed that “68% greater than average” density threshold, and begin to gravitationally collapse. Once you cross this threshold, your fate is sealed: you’re going to overcome the expansion of the Universe
In short order, or only another few million years, massive cold streams of gas will intersect, giving rise to not only the first stars, but possibly to the first seeds of supermassive black holes, via direct collapse, as well. Even though the Universe was only born with tiny, minuscule seed fluctuations — fluctuations that were predicted back when the Big Bang was first formulated back in the 1940s, but which are so small that they only were first detected with the advent of NASA’s COBE satellite in the 1990s — most of the regions that were born with large-magnitude overdensities will begin forming stars, proto-galaxies, and black holes within the first 200 million years of our cosmic history.

But these early structures, many of which have been shown to exist in surprisingly large numbers and early abundances by the JWST, cannot be relics from a previous stage of the Universe that represented a “collapsing” phase prior to the beginning of our hot Big Bang. If that were the case, the evidence that we see in the CMB would be vastly different, indicating enormous overdensities of very great magnitudes on small angular scales: something that would have shown up in the data, but very clearly isn’t there.
You might also wonder about fluctuations on scales larger than the cosmic horizon: superhorizon fluctuations. Is it possible that, on some grander scale than our observable Universe, that there’s a region of space that includes us that is ~68% (or more) denser than the cosmic average? If so, that could imply that even though the portion of our Universe that we see is expanding, there’s an inevitable collapse that’s ahead of us in the future, as this grander region will eventually collapse, taking us and our entire visible cosmos with it. This is also a nice idea, but it’s again contradicted by the data: we see evidence for these superhorizon fluctuations — most strongly in the polarization signal from the CMB — and they are also small in magnitude: of about that same 1-part-in-30,000 that inflation predicts. Again, this is far too small to cause “collapse” instead of expansion.
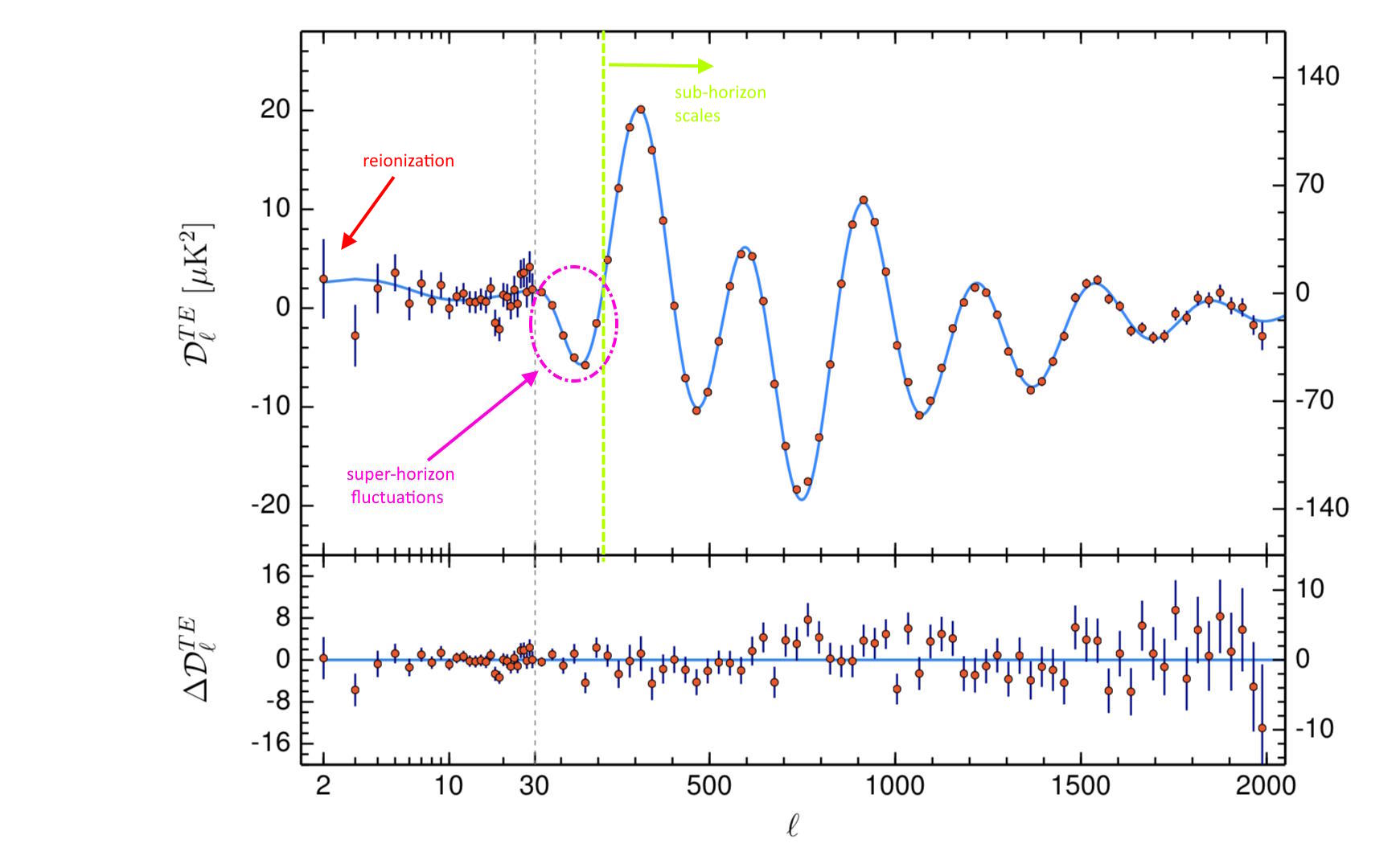
The overall lesson is that the question of “expansion vs. collapse” isn’t just for the observable Universe as a whole, but rather applies to each individual distance scale and region of cosmic volume that we can consider. So long as that volume of space remains near enough to the average cosmic density, the expansion will continue. (And, furthermore, with the onset of dark energy domination in our Universe billions of years ago, the prospects for the future growth of these structures is ever-diminishing.)
However, if the density of a particular region has grown large enough fast enough, and the density exceeds the average cosmic density by a critical amount — an amount of ~68% or so, that, when you think about it, really isn’t all that tremendous — then your space ceases to expand in that region, and instead becomes a bound state: capable of contracting or collapsing, but certain never to expand again. That’s the real race we need to consider: how a region of volume in the Universe evolves, and whether it gravitationally grows quickly enough to become gravitationally bound. If so, it can contract and will cease to expand; if not, expansion will continue unabated forever and ever.
The lack of large overdensities on large scales is unsurprising, as the largest bound structures that we confidently know of are all less than ~2 billion light-years in diameter, as opposed to the entire observable Universe, which spans more than ~93 billion light-years across. On the largest scales of all, the expansion is relentless and unavoidable. However, the cosmic structure that we see gives us strong evidence that the expansion doesn’t occur everywhere: just in between the bound structures that do form. When it comes to the question of cosmic expansion vs. contraction, it’s not an all-or-nothing proposition, but rather the answer is scale-and-magnitude dependent.
Send in your Ask Ethan questions to startswithabang at gmail dot com!