Ask Ethan: How do we know the Universe’s temperature?
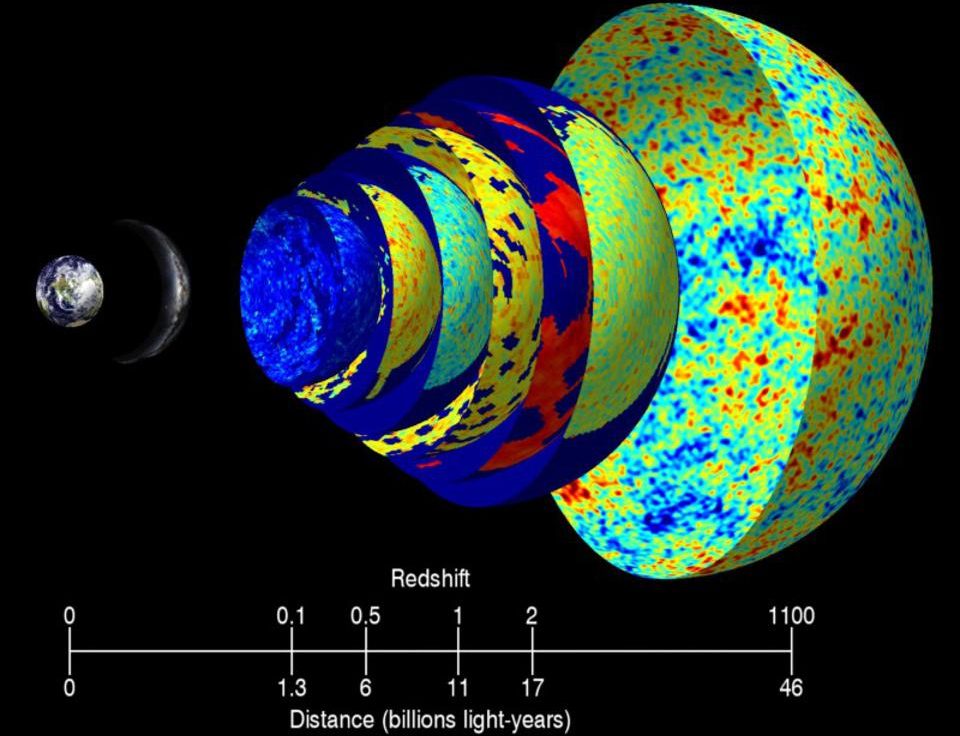
- From measuring the temperature of the radiation left over from the Big Bang, observable today as the cosmic microwave background, we infer that the Universe is just a few degrees above absolute zero: 2.725 K.
- However, that’s not the only source of energy in the Universe, and doesn’t even make up most of it; it represents less than 1% of the total energy in the Universe.
- And yet, that still provides the absolute best measurement of the Universe’s temperature. Here’s the science of why.
Whenever we want to determine what will happen to an object when we place it in an unfamiliar environment, we need to know a few properties about that environment. One of them, unflaggingly, is temperature. Whether something becomes solid, liquid, gas, or plasma depends on temperature. Changes in molecular structure are often temperature-dependent, and what you’re capable of observing or measuring is often dependent on “quieting” your system below a certain threshold of internal motion, a property that’s also temperature-dependent.
But what do we mean when we talk about the Universe’s temperature? That’s the question from Craig Schenck, who asks:
“[What is] the temperature of the Universe? This number is frequently referred to in cosmological discussions, and one often sees estimates of the temperature in degrees Kelvin… While I can see that the heat energy density of the expanding universe decreases with time, it’s not clear to me why the temperature of matter should change with expansion. What is the cooling mechanism, why does the mean kinetic energy of matter decrease, and where does it go? Or does the universe temperature merely refer to the CMB blackbody temperature, which is apparently not in equilibrium with all matter?”
It’s a fascinating question to explore, and how we found out the answer taught us a tremendous amount about what truly “matters” for the temperature of the Universe.

Credit: NASA, ESA, and J. Kastner (RIT)
What is temperature?
This is a tricky question, because we colloquially think about high temperatures as meaning “it’s hot” and low temperatures as meaning “it’s cold.” But in reality, “hot” and “cold” are measures of heat, while temperature is really a measure of how the total amount of heat is distributed among the particles in a given system within a volume of space. This might seem like splitting hairs, but when it comes to space, the difference becomes very important.
For example, if you traveled higher and higher in Earth’s atmosphere while wearing no protection, you would start to feel colder and colder. Normally, on Earth’s surface the ambient air around you exchanges heat with your body through molecular collisions. The more frequent and the more energetic those collisions are, the more energy they transfer into your body, while the less energetic those collisions are, the more the molecules of your body transfer energy into the air.
As you go to higher altitudes, the density of the air drops, and so does the pressure. With less frequent collisions, and more rarefied air, you’d expect to feel progressively colder, and the temperature will drop.
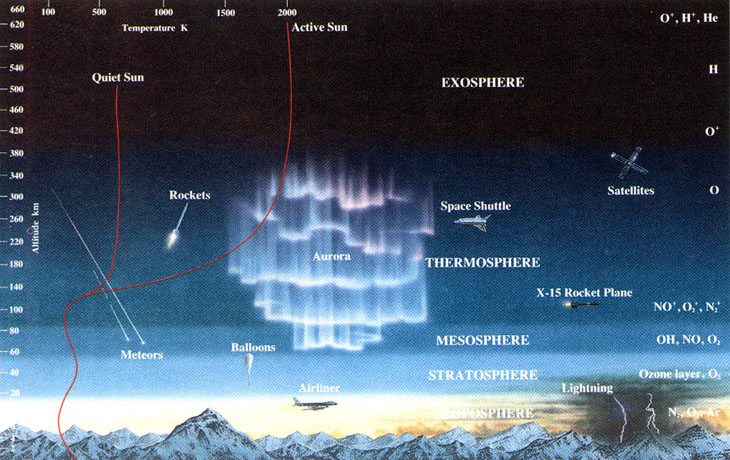
Credit: NASA/Smithsonian Air & Space Museum
That’s only partly true, however. Yes, you will continue to feel colder and colder, and the temperature will begin to drop as you rise to higher altitudes. But once you reach about 20 kilometers (or 12 miles) in altitude, the air temperature suddenly rises again! Yes, the density still drops, the pressure still drops, and most importantly, a human being will lose heat to the external environment more quickly. But the temperature increases.
The reason the temperature goes up is because, with fewer particles at that altitude to carry that heat, the heat energy that is present gets distributed across a much smaller number of molecules. Therefore, collisions between those molecules is less frequent, collision between molecules and whatever you put in that environment are less frequent, and the collisions that do occur don’t impart very much total energy to whatever’s in that environment.
At these low pressures, any object with a significant amount of heat will radiate that heat away more quickly than it can absorb it from the environment. At about 50 km of altitude, the temperature drops again, reaching a minimum at about 85 to 100 km, and then increases tremendously at altitudes above that. Without protection, a human at that altitude would freeze to death, despite the fact that temperatures there are even hotter than on Earth’s surface. The motion of molecules is a good way to measure temperature, but that’s not the same as total heat.

Credit: denisismagilov
Where does the Universe’s energy come from?
This is a question that you’d think would be easy to answer: just measure-and-calculate how much energy is in each different component of the Universe and compare them with one another. This has been a longstanding quest for people who study cosmology, as the ratios of the different forms of energy in the Universe determine how the Universe has expanded over its history and how it will expand into the future. Today, our best answer to that question is that the Universe is made of:
- ~0.01% photons,
- 0.1% neutrinos,
- 4.9% normal matter,
- 27% dark matter,
- and 68% dark energy,
along with only upper limits on the amount of energy that could exist in any other forms.
However, not all of that energy is useful energy, in the sense that it isn’t able to transfer it from one component into another. Dark energy behaves as a form of energy inherent to space itself, and it’s uniform in all locations so it cannot be transferred into any object placed in an arbitrary location in the Universe. Dark matter is made up, in theory, of particles in motion. But because those particles don’t collide or exchange energy and momentum with normal matter — what we make solid objects out of — it can’t heat up or increase the temperature of such objects.

Credit: Ralf Kaehler/SLAC National Accelerator Laboratory
Similarly, neutrinos are incredibly inefficient at transferring energy into or out of the normal matter that we know of; only in incredibly dense environments and at high energies, where nuclear physics processes copiously take place, can neutrinos make a substantial difference in the internal energy of an object. While that makes them very efficient at, say, carrying energy away from a supernova explosion, it makes them terrible at transferring energy into an arbitrary structure composed of normal matter.
That leaves only photons and normal matter as candidates to consider for injecting energy into another object in the Universe. If you were to put an object somewhere in space, you can imagine that it’s going to either heat or cool until it reaches what we call an equilibrium state: where the energy that it emits, in all forms, is equal to the cumulative amount of energy that it absorbs. Objects can absorb energy through collisions, either with photons or with matter particles, while they can emit it through collisions and through radiating it away.
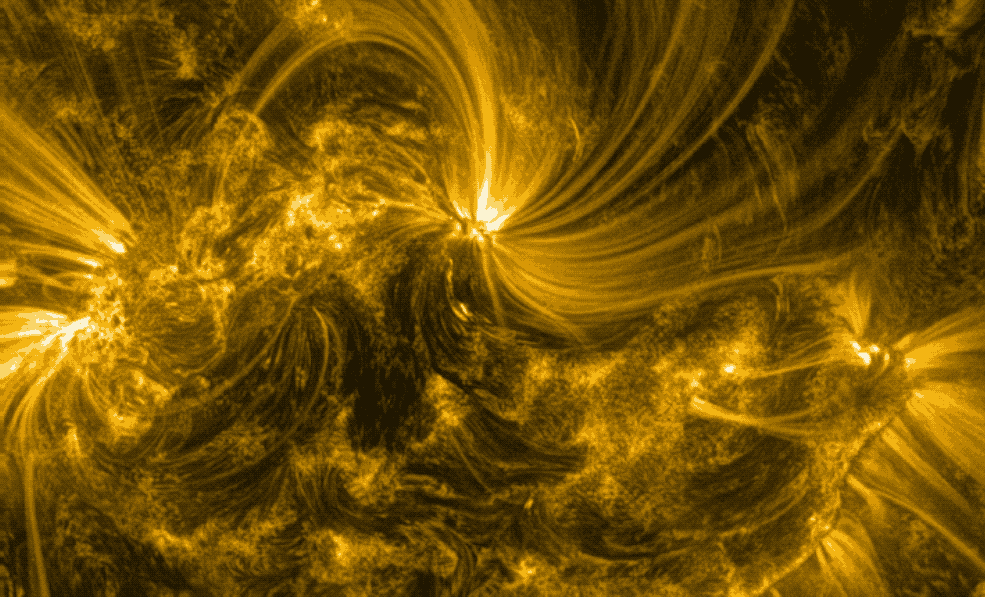
Credit: NASA/SDO
So, what is the right question to ask?
This is where we have to get quantitative. If you were to put an object out into the Universe, it would either heat up or cool down until it was in equilibrium with its surroundings. We therefore need to know what the various ways that energy gets transferred into objects. There are four major ways this can occur.
- There are photons flying in all directions throughout the Universe, and this has been the case ever since the onset of the hot Big Bang. Wherever you go in the Universe, so long as nothing shields you from this omnidirectional bath of radiation, this radiation exists; today, there are ~411 of these photons in every cubic centimeter of space.
- There are photons coming from other sources as well: stars, brown dwarfs, hot gas, and normal matter that radiates energy away. These photons aren’t uniformly distributed, but are localized to wherever you have normal matter with the appropriate properties: within galaxies.
- There are high-energy particles emitted by astrophysical objects like stars and stellar remnants. The solar wind and winds from other stars, the centers of galaxies, and cosmic particles that get accelerated by white dwarfs, neutron stars, and black holes are all included in this category.
- And finally, there are the particles found throughout the Universe — dust particles, gas particles, plasma particles, etc. — that dominate their environments. If you place another object in that environment, collisions between those particles and the particles making up your object can exchange energy until an equilibrium condition is reached.
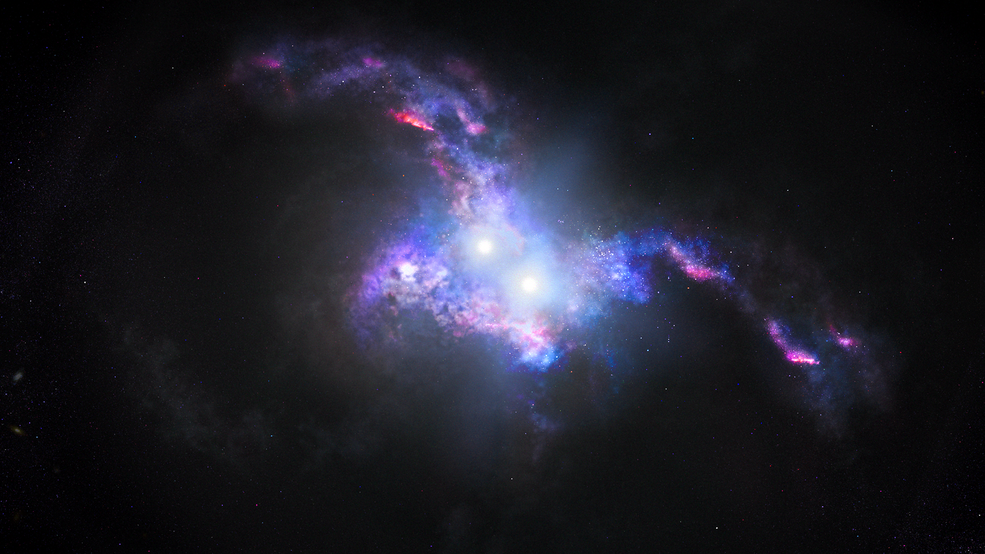
Credit: NASA, ESA, and J. Olmsted (STScI)
The right question to ask, then, is “which process dominates over most of the Universe?”
Extremely close to high-energy sources, the second and third processes will dominate, as a combination of particles and radiation emitted from these sources will heat other objects in that environment to very high temperatures and energies. However, those sources are very localized, representing only a tiny fraction of the volume of the Universe.
Wherever you have dense clumps of matter, the fourth process will dominate, as the energy in those collections of particles can easily transfer into whatever object you place in there. However, this is restricted to gas-rich, plasma-rich, or dust-rich regions, which are preferentially collected into galaxies. But the volume of space that exists between galaxies dwarfs the volume of space that galaxies occupy, even if we include the clouds of gas that populate the halos of galaxies. The depths of intergalactic space are simply too great. The temperature might be large where we are, dominated by the Sun, and it might be smaller (but still large compared to intergalactic space) in the Milky Way’s interstellar medium. But neither of these locations are representative of the majority of the Universe.
That only leaves three candidates for where most of the Universe’s energy comes from:
- the photons left over from the Big Bang
- the photons produced by other processes, like stars and other radiating forms of matter
- the energy of particles that permeate intergalactic space
If we can quantify the energy from these three sources, we can meaningfully answer this question: “If we put an object into the depths of intergalactic space, and it comes to equilibrium with its environment, what will its temperature be?”

Credit: ESO/INAF-VST/OmegaCAM. Acknowledgement: OmegaCen/Astro-WISE/Kapteyn Institute
The answer: the temperature of the Universe.
So, which of those three remaining candidates is the dominant one? It’s hard to know without doing the calculation. On the one hand, matter particles are very massive, and even slow-moving particles can carry lots of kinetic energy. On the other hand, the Universe is old and full of stars, stellar remnants, and supermassive black holes, all distributed for billions of light-years across the visible Universe. On the third hand, because there are three things we’re deciding between (and we won’t let the restrictions of human anatomy stop us from proceeding with this analogy), there are a tremendous number of photons that were produced in the hot Big Bang; even though they’re very low in energy today, a large number of low-energy quanta can carry more total energy than a small number of high-energy ones.
As the Universe expands, the number density of particles dilutes because the total number of particles stays constant while the volume increases. Whenever a photon gets absorbed by matter in the Universe, that matter heats up, but it will also re-radiate photons until its back in equilibrium with its surroundings.
However, the wavelength of each individual photon stretches as the Universe expands. Remember that it’s the wavelength of a photon — from crest to trough, to crest again — that defines its energy. As the Universe expands, the wavelength stretches, and so each individual photon loses energy as it travels through the expanding Universe. Even though photons outnumber the matter particles in the Universe by more than one billion to one, you might think that this means matter particles will eventually win.

Credit: Larry McNish/RASC Calgary
But that’s not true, either! Remember, matter can have its energy broken up into two parts: the rest-mass energy, which comes from Einstein’s E = mc2, and kinetic energy, which is the energy of its motion. The expansion of the Universe cannot touch the rest-mass part; that component remains just as constant today as it was when the Universe was only a fraction-of-a-second old. But the second part — the energy of a particle’s motion — gets stretched and lessened with the expansion of the Universe just as surely as a photon’s wavelength gets stretched.
You can visualize this in one of two ways.
- You can remember that just as a photon has properties of both a particle and a wave, so does matter — in the form of its quantum mechanical de Broglie wavelength. As the Universe expands, that wavelength gets stretched in precisely the same fashion as a photon’s does.
- You can imagine that a particle is emitted by object A and heads toward object B at a certain speed. However, as the Universe expands, the distance between object A and object B increases, and so the amount of time it takes to go from A to B increases as well. The longer it takes to reach object B, the slower it will appear to be moving when it arrives.
So then, the only options for what determines the temperature of the Universe come in the form of light: either light from astrophysical objects or light from the Big Bang. How do we decide? We measure the background light from the Universe and see which explanation fits better.

Credit: Sch/Wikimedia Commons (L); COBE/FIRAS, NASA/JPL-Caltech (R)
If the leftover light from the Big Bang dominates the Universe’s energy content, then the spectrum of the light we see would be a perfect blackbody: as though it were heated up to some high temperature, emitted light, and then that light was simply stretched by the expansion of the Universe. If, on the other hand, the light emitted from astrophysical objects dominated, including if it was absorbed and re-radiated by the matter in the Universe, then the spectrum of the light we see would instead be approximated by the sum of a series of blackbodies: just like the light from our Sun and all stars.
When we measure the light from the Universe, the answer is clear: It isn’t just a perfect blackbody, it’s the most perfect blackbody we’ve ever observed. It’s inconsistent with all explanations other than being light left over from the hot Big Bang. That’s why we know — in the deepest depths of intergalactic space — an object placed there would gain or lose energy until it reached the background temperature of that light left over from the Big Bang: 2.725 K.
If you’re in or very close to a large, dense clump of matter, such as within a galaxy, a group of galaxies, or a cluster of galaxies, your temperature will usually be greater than that, though if that matter expands quickly enough, as it does in the Boomerang nebula, it could also be colder than the cosmic average. But most of the Universe, by volume, is in the depths of intergalactic space. In these locations, it’s the radiation left over from the Big Bang that determines your temperature. A little less than three degrees above absolute zero may not be much, but then again, the Universe is a pretty cool place.
Send in your Ask Ethan questions to startswithabang at gmail dot com!