5 Reasons why the 21st century will be the best one ever for astrophysics
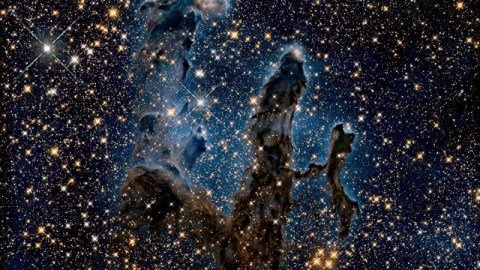
The 20th century held some incredible advances across all the science. But astrophysics’ best days are yet to come.
“When we have found how the nucleus of atoms is built up we shall have found the greatest secret of all — except life.” –Ernest Rutherford
It’s been a staple of science throughout the centuries: the arrogant thinking that we’ve almost arrived at the ultimate answers to our deepest questions. Scientists thought that Newton’s mechanics described everything, until they discovered the wave nature of light. Physicists thought we were almost there when Maxwell unified electromagnetism, and then relativity and quantum mechanics came along. And many thought the nature of matter was complete when we discovered the proton, neutron and electron, until high-energy particle physics revealed an entire Universe of fundamental particles. In just the past 25 years, five incredible discoveries have changed our understanding of the Universe, and each one holds the promise of an even bigger revolution. There’s never been a better time to look into the deepest mysteries of existence.
1.) Neutrino Mass. When we began calculating the neutrinos that should come from the Sun, we arrived at a number based on the fusion that must be occurring inside. When we measured the neutrinos coming from the Sun, we only saw a third of what we expected. Why? That answer came forth only recently, where a combination of measurements of solar and atmospheric neutrinos revealed that they can oscillate from one type into another, due to the fact that they have mass!
What it means for astrophysics: Neutrinos are the most abundant massive particles in the Universe: about one billion times as numerous as electrons. If they have mass, they do the following:
- make up a fraction of the dark matter,
- fall into galactic structures at late times,
- possibly form a strange astrophysical state known as a fermionic condensate,
- and may have a connection to dark energy.
Neutrinos, if they have mass, may also be Majorana particles (rather than the more common Dirac-type particles), which may enable a new kind of nuclear decay. They may also have ultra-heavy, left-handed counterparts which could explain the dark matter. Neutrinos also are responsible for carrying a way a large fraction of energy in supernovae, are responsible for how neutrons stars cool, affect the Big Bang’s leftover glow (the CMB), and will remain an interesting and potentially important part of modern cosmology and astrophysics.
2.) The Accelerating Universe. If you begin the Universe at the hot Big Bang, it has two vital properties: an initial expansion rate and an initial matter/radiation/energy density. If the density were too great, the Universe would recollapse; if it were too small, the Universe would expand forever. But in our Universe, the density and expansion are not only perfectly balanced, but a tiny amount of that energy comes in the form of dark energy, which means our Universe begins accelerating after about 8 billion years, and has continued to do so ever since.
What it means for astrophysics: For the first time in human history, we actually have some insight into the fate of the Universe. All the objects that are not gravitationally bound together will eventually accelerate away from one another, meaning that everything beyond our local group will eventually accelerate away. But what is the nature of dark energy? Is it truly a cosmological constant? Is it related to the quantum vacuum? Is it a field whose strength changes over time? Upcoming missions, like ESA’s Euclid, NASA’s WFIRST satellite and the new 30-meter-class telescopes coming online will better measure dark energy and allow us to characterize exactly how the Universe is accelerating. After all, if the acceleration increases in strength, the Universe will end in a Big Rip; if it decreases and reverses, we can still get a Big Crunch. The very fate of the Universe is at stake here.
3.) Exoplanets. A generation ago, we thought there were likely planets around other star systems, but had no evidence to support that claim. At the present, thanks largely to NASA’s Kepler mission, we have found and verified thousands. Many solar systems are different from our own: some contain super-Earths or mini-Neptunes; some contain gas giants in the inner portions of the solar systems; most of the ones that contain Earth-sized worlds at the right distance for liquid water orbit around tiny, faint, red dwarf stars, not stars like our Sun. And yet, there’s so much more still to discover.
What it means for astrophysics: For the first time ever, we’ve identified worlds that are potential candidates for inhabited planets. We are closer than ever before to finding signs of alien life in the Universe. And many of these worlds may someday become homes for human colonies, if we so choose to go down that route. The 21st century will see us begin to explore these possibilities: to measure the atmospheres of these worlds and look for signs of life, to send space probes to them at a significant fraction of the speed of light, and to characterize them by their similarities to Earth in terms of oceans/continents, cloud cover, oxygen content in the atmosphere, and how much their land “greens” from summer to winter. If you’re curious about the truth that’s out there in the Universe, there’s never been a better time to be alive.
4.) The Higgs Boson. The discovery of the Higgs particle in the early 2010s completed, at last, the Standard Model of elementary particles. The Higgs boson has a mass of around 126 GeV/c2, decays after about 10–24 seconds, and has all the decays the Standard Model predicts it should. There are no signatures of new physics beyond the Standard Model at all in this particle’s behavior, and that’s a big problem.
What it means for astrophysics: Why is the Higgs mass so much less than the Planck mass? It’s a question that can be phrased differently: why is the gravitational force so much weaker than all the other forces? There are many possible solutions: supersymmetry, extra dimensions, fundamental excitations (the conformal solution), the Higgs is a composite particle (technicolor), etc. But so far, all of these solutions have no evidence supporting them, and boy, have we looked!
At some level, there must be something fundamentally new out there: new particles, new fields, new forces, etc. All of these will, by their nature, have astrophysical and cosmological consequences, and those effects are all model dependent. If particle physics, for example, at the LHC, doesn’t yield any new clues, it’s possible that astrophysics will! What goes on at the highest energies and on the shortest distance scales of all? The Big Bang — and also cosmic rays — brought us higher energies than any human-made accelerator ever will. The next clues to solving one of the biggest problems in physics may come from space, not from Earth.
5.) Gravitational Waves. For 101 years, this was the holy grail of astrophysics: searching for direct evidence of Einstein’s greatest unverified prediction. When Advanced LIGO came online in 2015, it achieved the sensitivity necessary to detect the ripples from the shortest-frequency, highest-magnitude gravitational wave sources in the Universe: inspiraling and merging black holes. With two confirmed detections under its belt (and more on the way), Advanced LIGO has moved gravitational wave astronomy from a possibility into a bona fide science.
What it means for astrophysics: All of astronomy, up until now, has been light-based, from gamma rays to visible light all the way into microwave and radio frequencies. But detecting ripples in spacetime is an entirely new way to view astrophysical phenomena in the Universe. With the right detectors at the right sensitivities, we’ll be able to see:
- neutron star mergers (and learn whether they create gamma-ray bursts),
- white dwarf inspirals and mergers (and to correlate them with Type Ia supernovae),
- supermassive black holes devouring other masses,
- gravitational wave signatures of supernovae,
- pulsar glitches,
- and, potentially, the leftover gravitational wave signature from the birth of the Universe.
Gravitational wave astronomy is in its infancy, but has just become a bona fide scientific field. The next steps are to increase sensitivity and frequency range, and to begin to correlate what we see in the gravitational sky with the optical sky. The future is on its way.
That’s not even counting some of the other great puzzles that are out there. There’s dark matter: the fact that over 80% of the mass in the Universe is completely invisible to both light and normal (atomic) matter. There’s the problem of baryogenesis: why our Universe is filled with matter and not antimatter, even though every reaction we’ve ever observed is completely symmetric between matter and antimatter. There are paradoxes related to black holes; there are mysteries and unknowns surrounding cosmic inflation; we have yet to construct a successful quantum theory of gravity.
There’s always a temptation to think that our best days are behind us, and that the most important and revolutionary discoveries have already been made. But if we want to comprehend the biggest questions of all — where our Universe comes from, what it’s truly made of, how it came to be, where it’s headed in the far future, how it will all end — we still have work to do. With unprecedented telescopes in size, range, and sensitivity set to come online, we’re poised to learn more that we’ve ever known before. There’s never a guarantee of victory, but every step we take brings us one step closer to our destination. No matter where that turns out to be, the journey continues to be breathtaking.
Ethan Siegel is the author of Beyond the Galaxy and Treknology. You can pre-order his third book, currently in development: the Encyclopaedia Cosmologica.