Origin of LIGO’s merging black holes finally discovered!

The massive black holes that formed LIGO’s first event were a surprise, and then a mystery. Here’s the long-awaited solution!
“Black holes can bang against space-time as mallets on a drum and have a very characteristic song.” –Janna Levin
In order to produce the gravitational wave signals that LIGO has seen so far, two extremely massive stars in a close, binary orbit must have both gone supernova an extremely long time ago. Over billions of years, those black holes spiraled into one another, as their orbits slowly decayed over the aeons, emitting small amounts of gravitational radiation at each step along the way. Finally, in the final fractions of a second, those ripples in spacetime were enough to vibrate our detectors here on Earth by less than a thousandth the width of a proton. That’s what it took to deliver our first directly detected gravitational wave signal, a century after Einstein’s relativity first predicted them.
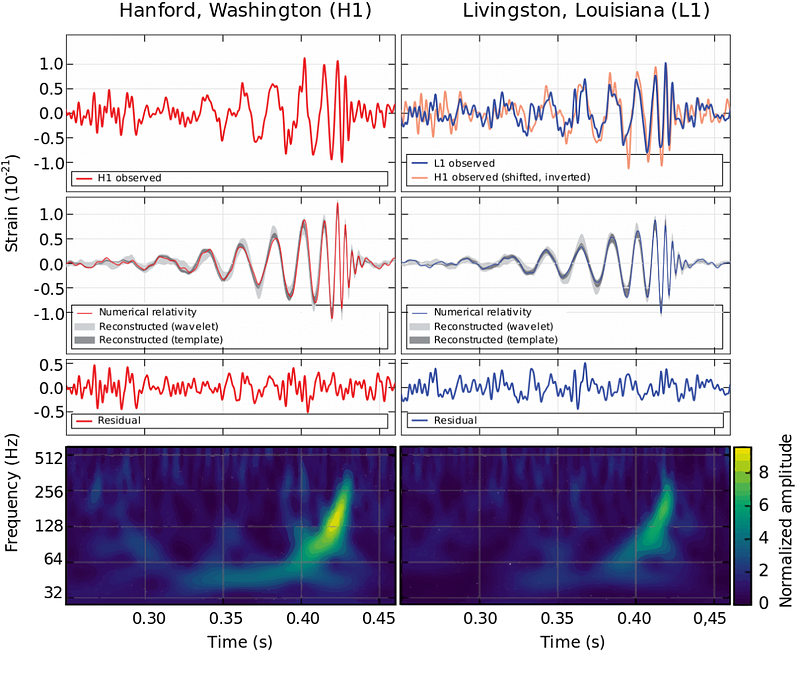
Before these gravitational waves were seen, all we had were theoretical models of what stellar-mass black holes might be. Contrary to the supermassive ones at the centers of galaxies, where we could measure the stars in orbit around them, the high-energy radiation emitted from the infalling matter, or the energy of the jets leaving them, all we had for these objects — the most common black holes in the Universe — was a story. We knew that stars that were massive enough would not only fuse hydrogen into helium during their main lifetime, and then turn into a red giant, fusing helium into carbon, but would go beyond that, heating up internally to achieve fusion reactions that less than 1% of stars will ever attain. Carbon fusion will begin, then oxygen, then silicon and sulphur and finally the core will be filled with iron, nickel and cobalt: elements too stable to fuse into heavier ones under normal conditions.
Stars need to be many times the mass of the Sun — at least 8-to-10 but perhaps even more — to reach this stage. At this point, the inner core of the star, since there’s no more fusion occurring, runs out of its prime source of radiation, which was the only thing holding the nuclei inside up against gravitational collapse. So the core of the star collapses, catastrophically, and implodes, giving rise to a Type II Supernova.
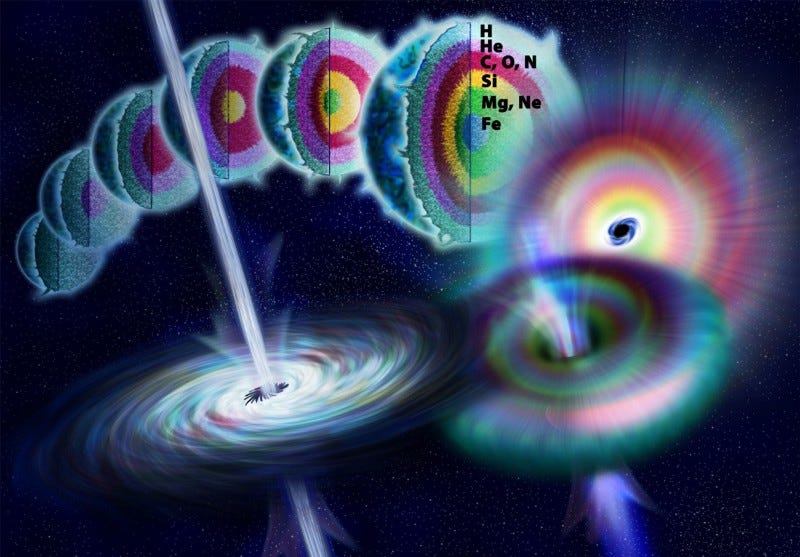
The thing is, a star needs to initially be very massive to make a black hole. The overwhelming majority of the star that gives rise to a supernova gets blown off by the explosion; it’s only the innermost core that collapses. Most of the stars that do collapse give rise to neutron stars, just two or three times the mass of the Sun. And the stars that give rise to black holes — the ones 20, 40 or more times the mass of our Sun — were expected to lead to black holes maybe between 5 and 10 solar masses. Maybe the most massive ones would be even 15 or 20 times the mass of our Sun.
But there’s a limit; high-mass stars tend to do something called quench star formation. The idea is that as a young star gets more and more massive, it burns brighter and hotter, and it not only prevents more matter from falling onto that star and growing it, it ionizes all the matter around it, and blows it off from the entire vicinity. In other words, it prevents all the other stars around it from growing larger; that’s what quench means.
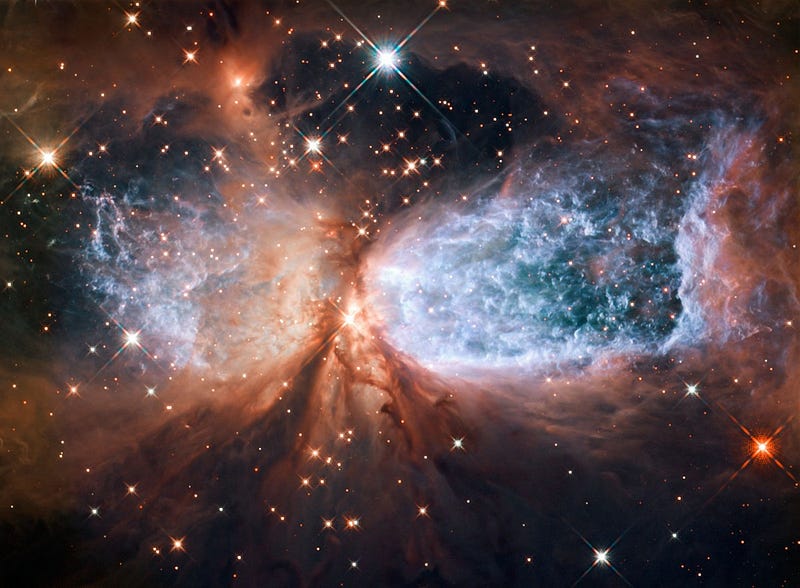
So for two stars to have lived, died in supernovae, and created both a 36 and a 29 solar mass black hole means that something had to happen to avoid this scenario. What actually happens, we think, is more peculiar than you might have imagined altogether. The stars that gave rise to the black holes couldn’t have been formed too late (or with too many heavy elements in them) according to numerical models, which indicate that most likely they had only about 10% of the heavy elements (carbon, oxygen and iron, for example) found in our Sun.
A new paper by Krzysztof Belczynski, Daniel E. Holz, Tomasz Bulik & Richard O’Shaughnessy, as well as a letter by J.J. Eldridge, suggest, based on simulations, that black hole binaries such as this arose in great numbers very early on in the Universe. Rather than from a Type II supernova, there are likely a whole class of binary black holes of ~30 solar masses (or slightly more) that arose from:
- massive binary star systems,
- between 40 and 100 solar masses to start,
- from when the Universe was only about ~2–3 billion years old,
- and that likely formed either in dwarf galaxies or on the outskirts of what would become a spiral galaxy: where there are fewer heavy elements.
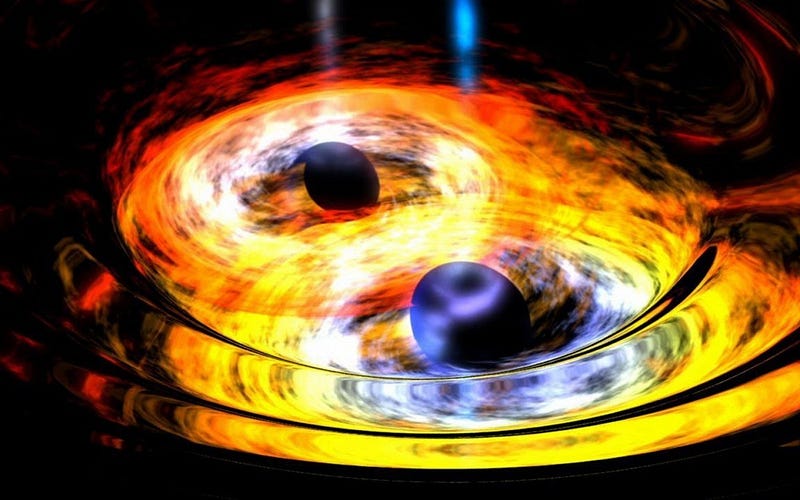
Over time, the radii of these stars increase as they heat up, making it easier to strip off their outer layers. The first one will go supernova as normal, but the second one will suffer a different fate. What happens in a binary system, rather than getting hotter and hotter and larger and larger, is that the outer layers get thrown off, via gravitational interaction, into the interstellar medium around them. The first black hole to form will also devour some of that material, but black holes are not very good eaters; they spit out most of what falls in. If both stars are massive and close enough, the second can lose its outer envelope. The core inside, then, simply contracts down and collapses without very much fanfare at all. In this way, we can get black holes without the standard, corresponding supernova explosions we know and recognize.
In addition, the “common envelope” phase shrinks their mutual orbit, bringing them closer and closer to merger status. Despite many years of research, the quantitative answer of how much these orbits shrink by is still an open question with very large uncertainties. Nevertheless, the simulations of Belczynski’s team indicates that these black hole binaries very likely formed more than 10 billion years ago, and their inspirals and merger occurred just 1.3 billion years ago, with the light reaching us today.
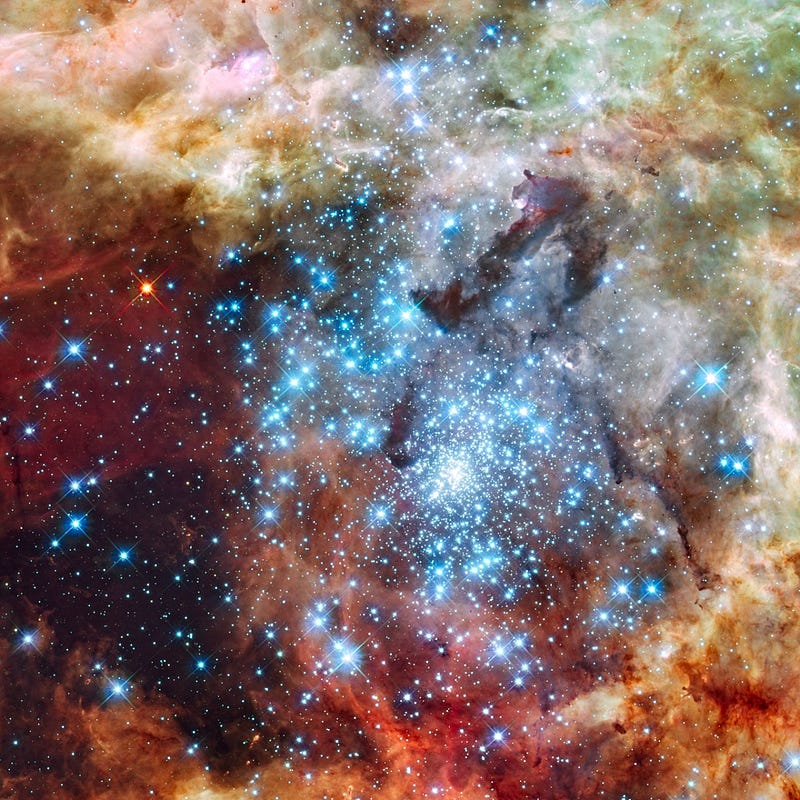
There is another possibility they entertain, however: a much younger, massive cluster of stars — with higher mass binaries inside — could have created these black holes much more recently. Perhaps clusters like the one inside the massive Tarantula Nebula in our own local group give rise to black hole binaries, and, with stars up to 260 times the mass of our Sun in there, perhaps ~30–40 times the mass of our Sun isn’t even as large as these black holes get. Regardless of their origin, which we should be able to figure out as more statistics and detections come in, the next generation of gravitational wave observatories should be able to detect perhaps as many as 1,000 of these binary black hole mergers per year. We’re entering, for the first time, the era of direct black hole astronomy, courtesy of gravitational waves. What it means for astrophysics is more than most of us ever anticipated.
This post first appeared at Forbes, and is brought to you ad-free by our Patreon supporters. Comment on our forum, & buy our first book: Beyond The Galaxy!