Why Measuring Redshifts Isn’t Enough To Understand The Universe
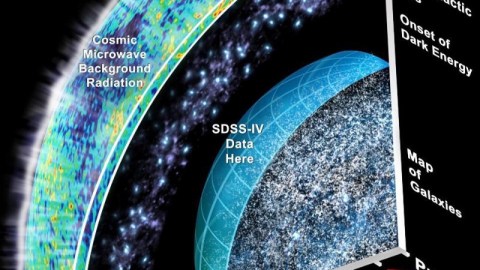
“Hubble’s Law” is only an approximation, and breaks down when we need it most.
From anywhere in the Universe, you can choose to look out at any other galaxy that’s present. When you do, you’ll find that the farther away it is from you — at least, on average — the longer the wavelength of that light is: it’s all shifted towards the redder end of the electromagnetic spectrum. This relationship, between the distance to a faraway object and its redshift, is at the heart of modern cosmology and was the first piece of evidence to reveal the expanding Universe and hint towards the Big Bang: the earliest moments of our Universe.
But measuring a distant object’s redshift, as powerful a tool as it is, isn’t enough to tell us everything we’d want to know about the Universe. In fact, if all we relied upon were redshift measurements to inform us about the Universe, we’d not only severely mis-estimate the distances to galaxies throughout the Universe, but we’d catastrophically fail to understand what the Universe looks like. There’s a lot more going on in the Universe than just mere expansion, and figuring out how the Universe responds to all of the processes happening inside is the key to extracting meaningful information about what’s truly going on.
In cosmology, just like in any other science, we frequently begin our investigations with a picture of reality that’s largely correct, but also oversimplified. On the largest scales, we assume that the Universe is isotropic, or the same in all directions, and homogeneous, or the same in all locations. To an extraordinary level of accuracy, this is true: if you were to draw a “box” around any region of the Universe that was large enough — perhaps a few billion light-years on each side — you’d find that, to greater than ~99.99% consistency, every region of the Universe was identical. On average, there would be only very slight deviations in the temperature, density, and overall motion of every object in the Universe.
But the Universe isn’t coarse-grained like that at all. When we take measurements of objects in the Universe, we generally measure things one source at a time: each galaxy, quasar, or other luminous object has data that we collect from it all on its own. This is a problem, because the scales of an individual galaxy are much, much smaller than this scale of uniformity: at significantly less than one million light-years in any direction, each galaxy represents a region of the Universe whose density is much greater than the large-scale average.
The reason for this is simple: even though the Universe starts off incredibly uniform, it’s had 13.8 billion years for gravitation to do its thing. Yes, the Universe is expanding the entire time, with the expansion rate gradually changing as the Universe’s density drops. Matter gets less dense as the volume increases, while radiation not only dilutes but loses energy as it travels through the expanding Universe, as its wavelength — which defines its energy — gets stretched to a longer, redder state.
Even though the Universe came into existence with only tiny imperfections to its uniformity, with deviations of just ~0.003% from the mean value, on average, gravitation is relentless and cosmic timescales are incredibly long. Even as the Universe expands, the initially overdense regions work to draw more and more of the surrounding matter into them, while the initially underdense regions do the opposite: preferentially giving up their matter to the more dense regions surrounding them. Over millions, and even billions, of years, matter begins to clump and cluster together, forming stars, galaxies, galaxy groups and clusters, and even, on the largest scales, the great cosmic web.
In between these matter-rich structures are enormous cosmic voids: regions with less matter than average, often spanning hundreds of millions or even billions of light-years in diameter. As matter coalesces into these individually bound structures like stars and galaxies, those individual structures find themselves being drawn into the larger-scale gravitational imperfections that grow into enormous collections of matter: galaxy clusters. Within these galaxy clusters, many thousands of large, massive galaxies can often be found, confined to volumes barely larger than that possessed by our own Local Group: just a few million light-years across.
Looking at the individual galaxies within a large group or cluster of galaxies illustrates the problem of looking at redshift-versus-distance as an absolute law: it isn’t one. In the Virgo Cluster of galaxies, which is the closest major cluster to our own Milky Way at just 50–60 million light-years distant, the problem immediately becomes apparent. On average, based on how quickly the Universe appears to be expanding, we expect the galaxies within the Virgo Cluster to be moving away from us at ~1000 km/s. But when we look at the individual galaxies themselves, there’s an enormous range of speeds: some receding at speeds greater than 2000 km/s, with more than double the expected redshift, and some that are even moving towards us rather than away from us, with their light blueshifted instead of redshifted.
This is something that should be expected, because of precisely the fact that the Universe isn’t precisely uniform on all — particularly small — cosmic scales. If you were to “drop” a mass at rest from just outside of the Solar System, it would be gravitationally attracted to all of the masses within it, dominated by our Sun. Although it would begin both moving and accelerating slowly, over time, it would pick up steam: just like every long-period comet that takes the plunge into our inner Solar System. By the time it reached perihelion, its closest approach to the Sun, gravitation alone could cause this mass to accelerate from “being at rest” to speeds greater than ~600 km/s, or approximately 20 times the Earth’s speed in its orbit around the Sun.
Now, if you were far away from the Solar System and you chose to measure the light from this mass, as well as the light from the Sun, what would you find?
The Sun, relative to small masses like this imagined plunging one, is largely stationary: the redshift you measure for it will depend on the combination of its peculiar motion relative to you — i.e., how much of a cumulative gravitational tug it’s been given by everything that’s ever tugged on it — and the effects of the Universe’s expansion. But for the small mass, there’d be an extra shift, due to its motion, that corresponds to up to ±600 km/s in any direction. This motion could be towards you, away from you, or perpendicular to your line-of-sight.
Now, except for some very rare cases involving extremely fast-moving objects, short distances, and long-period observations, it’s only the line-of-sight motions that are actually measurable. When an object moves relatively towards you, its light appears blueshifted; when it moves relatively away from you, its light appears redshifted. But unless you’re capable of detecting tiny motions in the transverse direction, usually corresponding to positional differences of just a few million kilometers over ~year timescales at the distances of million or billions of light years, this line-of-sight motion is all you’ll be able to tease out.
For galaxies within a galaxy cluster, it’s a very similar situation. The individual galaxies are pulled towards the central region by the mutual gravitation of not only all the galaxies, stars, gas, dust, plasma, and black holes inside these galaxy clusters, but all of the dark matter as well. For large, massive galaxy clusters, these individual galaxies can zip around, inside, with additional speeds of up to multiple thousands of kilometers-per-second: a few percent of the speed of light at their fastest. Some of these galaxies, relative to your line-of-sight, will appear to be moving towards you while others are moving away from you. Even though all of these galaxies are located at approximately the same distance from you, they’ll display dramatically different redshifts from one another.
This is why measuring the redshift of any one individual object isn’t very reliable for telling you anything in particular about the Universe. For any object you’re measuring, you aren’t just observing its cosmological redshift — the redshifting of light due to the expansion of the Universe — but also a combination of the object’s local motion through the Universe, influenced by the sum total of all the gravitational effects it’s experienced, all relative to your own motion as well. The idea that the Universe is the same everywhere is only true on average; for any individual object, there are deviations, due to local effects, that make it very dangerous to draw larger conclusions about the Universe.
But when you look at the aggregate effects of what happens to many, many galaxies, you find something else: your overall view of the Universe gets distorted. Wherever you have a large amount of mass in one location in space — a large-scale overdensity like a galaxy cluster, cosmic filament, or something even larger — it’s going to impart large peculiar velocities, including along the line-of-sight, to every mass that’s bound to it. With many observable objects all at the same distance, but with widely-varying redshifts, mapping out objects becomes challenging.
You might expect that you’d be able to simply map out objects by their redshift:
- you measure a redshift,
- you know Hubble’s law and how the expanding Universe works,
- so you can assign a distance to each object,
- and create a map of the Universe.
This is the precise recipe for seeing a distorted Universe that doesn’t take these gravitational effects into account. The first plots, in fact, that saw this effect led to a very catchy name for these redshift-space distortions: Fingers of God, because they point at you.
When large-scale structures are in the process of assembling — which we can see when we look back early enough — large numbers of galaxies can fall in coherently, such as when a smaller galaxy group gets absorbed into a cluster. This also leads to a redshift-based distortion that artificially puts large numbers of galaxies all at the same, systematically shifted (i.e., incorrect) distance, creating the opposite effect: Pancakes of God, which are compressed in the direction that points towards you.
Thankfully, we aren’t stuck with redshifts alone, but have gotten good at mapping out the gravitational field of the Universe in a way that allows us to correct for this, bringing us from “redshift space,” which is easy to measure but is physically unrealistic, to “real space,” which attempts to assign accurate 3D positions to each individual object that’s out there. This is vitally important, because if we want to know what type of structure formed in the Universe — which itself depends not only on gravitation, but also what the Universe is made up of and what sorts of initial conditions the Big Bang began with — it’s important to know that our maps are reliable.
Over the past four decades or so, we’ve been able to collect enormous catalogues of data concerning how galaxies clump and cluster together in the Universe, creating the most precise and comprehensive maps of our cosmos’s large-scale structure ever. We can then use those maps, our knowledge of physics, and the inferred 3D distances between each and every pair of galaxies to infer all sorts of properties about our Universe. How much matter is present, both normal and dark, how fast the Universe is expanding, and whether there’s any spatial curvature or not can all be revealed through exactly this type of study.
If we want to understand what’s present in the Universe and how our cosmos has evolved from the Big Bang until now, measuring the large-scale structure of the Universe can be an invaluable tool. By seeing the types of structures that galaxies form, how they clump and cluster together, and how likely you are (or aren’t) to find galaxies separated from one another by a particular distance, you can reconstruct what the Universe is and isn’t made of, how it’s expanded over its history, and a host of other intrinsic properties to it.
But if all you did was measure how the light from each distant object was redshifted, you’d bias your answers severely, and would draw the wrong conclusions about the Universe. On cosmic scales, the fact that masses attract other masses leads to galaxies possessing rapid motions within the bound structures that they’re a part of, stretching them out along our line-of-sight if we look at redshifts and blueshifts alone. A vitally important part of understanding what our cosmic web actually looks like hinges on our ability to translate our data from what we observe into what’s actually there: from redshift space into real space. Although it’s tremendously difficult work, the reward — of getting to know the Universe as it actually is — is a commensurate payoff.
Starts With A Bang is written by Ethan Siegel, Ph.D., author of Beyond The Galaxy, and Treknology: The Science of Star Trek from Tricorders to Warp Drive.