Where is the Cosmic Microwave Background?
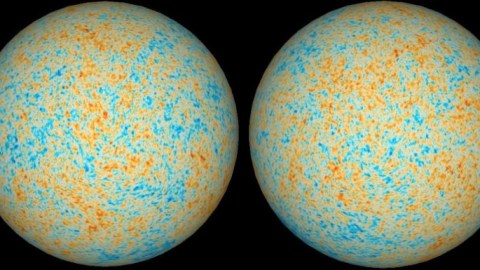
We claim it’s the leftover glow from the Big Bang, but where is this light actually coming from?
“We are told to let our light shine, and if it does, we won’t need to tell anybody it does. Lighthouses don’t fire cannons to call attention to their shining- they just shine.” –Dwight L. Moody
When you look out at the distant Universe, you’re also looking back in time, thanks to the fact that the speed of light — although huge — is finite. So if you look back at the farthest thing you can see, at the very first light visible to our equipment, you’re bound to reach something. In our Universe’s case, to the best of our knowledge, that’s the leftover glow from the Big Bang: the cosmic microwave background (CMB). But it’s possible that the Universe is infinite; there’s no reason to believe that the CMB we see is the edge or boundary in any way. So where, exactly, is the CMB?
Let’s start with the Big Bang itself, so we can put the CMB in perspective, and go from there. When the hot Big Bang first began — after a period of cosmic inflation that lasted for an indeterminate amount of time — the Universe had the following properties:
- It was large: most likely much, much larger (by at least factors of many hundreds) than the part of it that makes up our observable Universe.
- It was incredibly uniform — of the same energy density everywhere — to better than 1 part in 10,000 on average.
- It was tremendously hot. Take the highest energies reached at the Large Hadron Collider and raise it by at least a factor of 10,000,000; that hot.
- It wasn’t just hot, but dense as well. The densities of radiation, matter, and antimatter were trillions upon trillions of times denser than a uranium nucleus.
- And also, it was expanding incredibly rapidly, cooling as it expanded.
That was the Universe we started off with. That was our past, some 13.8 billion years ago.
But as the Universe expanded and cooled, some incredible things happened in our cosmic history, and they happened everywhere at once. The unstable matter/antimatter pairs would annihilate away when the Universe cooled below the temperature necessary to spontaneously produce them. Eventually, we were left with just a small amount of matter, which was somehow produced in excess over the antimatter.
As the temperatures continued to cool, nuclear fusion would occur between the protons and neutrons, giving rise to heavier elements. Although it took a significant amount of time — between three and four minutes (a lifetime in the early Universe) — for the formation of deuterium, the first step (one proton and one neutron make a deuteron) in all nuclear chain reactions, to stably occur, once it does we wind up with significant amounts of helium in addition to hydrogen, as well as trace amounts of lithium.
The first heavy elements in the Universe are formed here, amidst a sea of neutrinos, photons, and ionized electrons.
Now, it takes energies on the order of many MeV (or Mega-electron-Volts) to fuse light elements into heavier ones, but if you want to form neutral atoms? You need your energies to drop below just a few eV (or electron-Volts), about a factor of a million lower in temperature.
Forming neutral atoms is incredibly important if you want to see what’s going on, because no matter how much light you have, if you’ve got a whole bunch of dense, free electrons floating around, that light is going to scatter off of those electrons via a process known as Thomson (or, for high energies, Compton) scattering.
As long as you’ve got a high enough density of free electrons, all that light, pretty much regardless of energy, is going to bounce around, exchanging energy, and having whatever information was encoded destroyed (or, more accurately, randomized) by these collisions. So until you form neutral atoms, and “lock up” these free electrons so that the photons can travel unimpeded, you can’t really “see” anything. (Not with light, anyway.)
As it turns out, the Universe needs to cool below a temperature of around 3,000 Kelvin for this to happen. There are so many more photons than electrons (by about a factor of a billion) that you need to reach these “insanely low” temperatures just so that the highest energy photons — the one-in-a-billion that have enough energy to ionize hydrogen — dip below that critical energy threshold. By time this occurs, the Universe is around 380,000 years old, and the process itself takes a little over 100,000 years total to happen.
Now, this takes place everywhere at once, gradually (as we just covered), with all the light in the Universe finally free to stream outward, at the speed of light, in all directions. The CMB was emitted when the Universe was around 380,000 years old, and it wasn’t “microwave” light when it was emitted: it was infrared, with portions of it hot enough that it would have been visible as reddish light to human eyes, had there been any humans around at the time. We actually have sufficient evidence that the CMB’s temperature was hotter in the past; as we look to higher and higher redshifts, we see exactly this effect.
Extrapolating all the way back from what we observe today, a 2.725 K background that was emitted from a redshift of z = 1089, we find that when the CMB was first emitted, it had a temperature of about 2,940 K. The CMB is not at the edge of the Universe, but rather represent the “edge” of what we can see, visually. When we look out at the CMB, we find fluctuations in it as well: the regions of overdensity (which are coded “blue”, or cooler) and underdensity (which are coded “red,” or hotter), that represent the slight departures from perfect uniformity.
This is a good thing, for two reasons:
- These fluctuations were predicted by inflation, and were predicted to be scale-invariant. This was back in the 1980s; the observation and confirmation of these fluctuations by satellites in the ’90s (COBE), ’00s (WMAP) and ’10s (Planck) have verified what inflation dictates.
- These fluctuations, of overdense and underdense regions, are necessary to give rise to the patterns of large-scale structure — stars, galaxies, groups, clusters, and filaments — all separated by vast, cosmic voids.
Without these fluctuations, we’d never have a Universe that matches what we observe ours to be.
And yet, although the light from the CMB always originates from when the Universe was 380,000 years old, the light that we observe, here on Earth, is constantly changing. You see, the Universe is some 13.8 billion years old, and while the dinosaurs — had they build microwave/radio telescopes — could have observed the CMB for themselves, it would have been slightly different.
It would have been a few milliKelvin hotter, because the Universe was younger some hundred million years ago, but more importantly, the patterns in the fluctuations would have been entirely different from the pattern we see today. Not statistically, mind you: the overall magnitude and spectrum of hot-and-cold spots would be extremely similar (within the bounds of cosmic variance) to what we see today. But specifically, what’s hot today and cold today would be virtually unrelated to what’s hot-or-cold even one or two hundred-thousand years ago, much less hundreds of millions.
When we look out in the Universe, the CMB is there, everywhere, in all directions. It’s there for all observers at all locations, constantly being radiated towards everyone from what they observe as the “surface of last scattering.” If we waited around for long enough, we’d get to see not just a snapshot of the Universe as it was in its infancy, but a movie, that allowed us to map the overdensities and underdensities in three-dimensions as time went on! In theory, we can measure this far into the future, as the “microwave” background drops into the radio portion of the spectrum, as the photon densities drop from around 411-per-cubic-centimeter to tens, to single digits, all the way down to millionths of today’s density. The radiation will still be there, so long as we’re around to build big, sensitive enough telescopes to detect it.
So the CMB isn’t the end of the Universe, but rather the limit of what we can see, both distance-wise (as far as we can go) and time-wise (as far back as we can go). Until we can directly detect the signatures of what was released earlier — the cosmic neutrino background, gravitational waves from inflation, etc. — the CMB will be our window into the earliest time we can observe: 380,000 years after the Big Bang.
This post first appeared at Forbes, and is brought to you ad-free by our Patreon supporters. Comment on our forum, & buy our first book: Beyond The Galaxy!