What Do We Really Know About Dark Matter And Black Holes?
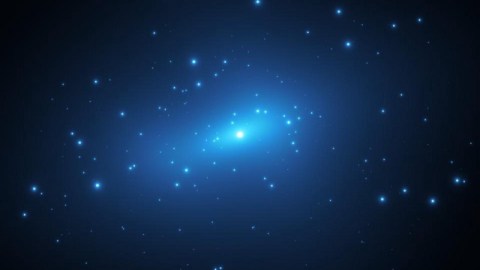
And what might we learn as we collect new, never-before-seen data?
If you took one of history’s top scientists from 100 years ago and dropped them into today’s world, what scientific revelations do you think would shock them the most? Would they be surprised to learn that the stars, which emit almost all of the light we see from the Universe beyond Earth, make up only a tiny fraction of the Universe’s mass? Would they be baffled at the existence of supermassive black holes, the most massive single objects in the Universe? Or would it be dark matter or dark energy that they found most puzzling?
It would be easy to understand their disbelief. After all, science is an empirical endeavor: our understanding of the natural world and Universe is informed primarily by what we observe and measure. It’s hard to fathom that objects or entities that emit no light of their own — that are not themselves directly observable through our telescopes — would somehow make up such a massive, important component of our Universe. And yet, almost every scientist working today has come to the same conclusion: our Universe is mostly dark. Here’s how we learned about it.
On the theoretical side, it’s important to recognize two separate things right from the start:
- theory tells us what to expect given certain conditions,
- but it also only tells us what’s possible in the Universe, not what our assumptions about the Universe’s conditions should be.
When Einstein put forth our modern theory of gravitation — General Relativity — it did something that no other theory did. It not only succeeded everywhere the prior (Newton’s) leading theory did, but it made a novel set of predictions that were distinct from that prior theory. It successfully explained the orbit of Mercury, which was an unsolved problem previously. It accommodated and included the observed facts of time dilation and length contraction. And it made novel predictions about the gravitational bending and shifting of light, which led to concrete observable consequences.
Just a few years after it was proposed, critical tests were performed, confirming the predictions of Einstein’s theory as matching our Universe and rejecting the null (Newtonian) hypothesis.
What Einstein’s General Relativity gives us is a framework for understanding the phenomenon of gravitation in our Universe. It tells us that, dependent on the properties and configuration of the matter and energy in the Universe, spacetime will curve in a particular way. The curvature of that spacetime, in turn, tells us how matter and energy — in all its forms — will move through that spacetime.
From a theoretical viewpoint, this gives us virtually limitless possibilities. You can concoct a Universe with any configuration you like, with any combination of masses and particles of radiation and fluids of various properties that you like, distributed however you choose, and General Relativity will tell you how that spacetime will curve and evolve, and how any components will move through that spacetime.
But it won’t tell you, on its own, what our Universe is made of or how our Universe is behaving. To know that, we have to inform ourselves by looking at the Universe we have, and determining what’s in it and where.
For example, we live in a Universe that has roughly the same amount of matter, on large scales, in all directions and at all locations in space. A Universe that has those properties — that’s the same in all locations (homogeneous) and in all directions (isotropic) — cannot be static and unchanging. Either the spacetime itself will contract, leading to a collapsed object of some type, or it will expand, with objects appearing to recede from us faster and faster the farther away from us they are.
The only way we know this to be true, however, is from our observations. If we didn’t observe the Universe and notice that the farther away a galaxy is from us, on average, the greater the amount that its light is redshifted, we wouldn’t have concluded that the Universe is expanding. If we didn’t see, on the largest scales, that the Universe’s average density was uniform to a 99.99%+ precision, we wouldn’t have concluded that it’s isotropic and homogeneous.
And in the places where, locally, enough matter has gathered in one place to form a bound, collapsed structure, we wouldn’t have concluded that there’s a supermassive singularity at the center if we didn’t have overwhelming observational evidence for supermassive black holes.
You might think of the famous image from the Event Horizon Telescope of this 6.5 billion solar mass behemoth at the center of Messier 87 when talking about supermassive black holes, but that’s just the tip of the metaphorical iceberg. Practically every galaxy out there has a supermassive black hole at their center. Our Milky Way has one that comes in at about 4 million solar masses, and we’ve observed it:
- indirectly, from stars moving around a large mass that emits no light at the galactic center,
- indirectly, from matter that falls into it and causes X-ray and radio emissions, including flares,
- and directly, with the same technology and equipment that measured the black hole at the center of Messier 87.
Many of us are hopeful that the Event Horizon Telescope collaboration will release an image of the Milky Way’s central black hole later this year. They have the data, but because it’s some ~1500 times less massive than the one we got our first image of, it changes on timescales that are ~1500 times faster. Producing an image that’s accurate will be a much greater challenge, especially given how faint this radio signal is in such a messy environment. Still, the team has expressed optimism that one will be forthcoming within the next few months.
The combination of direct and indirect evidence makes us more confident that the X-ray and radio emissions we are seeing from various sources throughout the Universe really are black holes. Black holes in binary systems emit telltale electromagnetic signals; we’ve discovered scores of them over the years. Active galactic nuclei and quasars are powered by supermassive black holes, and we’ve even observed them turning on and off as matter either begins or ceases to feed these central engines.
In fact, we’ve observed “radio-loud” supermassive black holes in a myriad of galaxies wherever we look. A new survey from the LOFAR array, for example, has begun surveying the northern celestial hemisphere, and with only a tiny fraction of the sky under their belt, they’ve already discovered more than 25,000 supermassive black holes. From a map of them, you can even see, already, how they clump and cluster together, following the large-scale distribution of massive galaxies in our Universe.
All of this discussion of black holes doesn’t even include the most revolutionary development of the past decade: the direct detections we’ve made using gravitational wave observatories. When two black holes inspiral and merge, they create gravitational waves: ripples in spacetime, a completely novel, non-electromagnetic (light-based) form of radiation. When those ripples pass through our gravitational wave detectors, they alternately expand and compress the space present in different directions, and we can see the patterns of those ripples in our gravitational wave data.
Right now, the only successful detectors we have are those under the guidance of the LIGO and Virgo collaborations, which are relatively small in scale. This limits the frequency of the waves they can observe, corresponding to low-mass black holes in the final stages of inspiral and merger. In the coming years, new, space-based detectors like LISA will take flight, enabling us to detect larger-mass black holes and to see them, and the smaller ones, long before the actual final moments of a merger occurs.
Meanwhile, there’s another enormous puzzle about our Universe: the dark matter problem. If we take into account all the matter that we know of and can directly detect — atoms, plasma, gas, stars, ions, neutrinos, radiation, black holes, etc. — it only accounts for about ~15% of the total amount of mass that must be there. Without about six times as much mass as we see, which cannot collide or interact the same way normal atoms do, we cannot explain:
- the fluctuation patterns seen in the cosmic microwave background,
- the large-scale clustering of galaxies and galaxy clusters,
- the motions of individual galaxies within galaxy clusters,
- the sizes and masses of galaxies that we observe,
- or the gravitational lensing effects of galaxies, quasars, or colliding galaxy groups and clusters.
Adding in just one new ingredient, some form of cold, collisionless dark matter, explains all of these puzzles in one fell swoop.
Yet, somehow, this is still dissatisfying in a sense. We know some general properties of what dark matter should be that, combined, all tell a compelling story about the Universe. But we have yet to directly detect whatever particle might be responsible for it. A species of matter that’s purely collisionless doesn’t necessarily explain the cosmic structure that appears on the smallest scales. It’s possible that there are purely gravitational effects — like dynamical heating — that are responsible for this mismatch, but it’s also more possible, and perhaps even more likely, that dark matter is not quite so simple.
Meanwhile, on the black hole side, we now see many supermassive black holes that are somehow grew to be a billion solar masses or more in just a few hundred million years: a tremendous puzzle for structure formation in our Universe. Based on our understanding of the first stars and how the earliest black holes would arise from them, we simply struggle to explain how they got to be so big so fast, as we see these behemoths at significantly earlier times than anticipated.
These are the frontiers of our knowledge, and represent some of the most pressing problems in modern cosmology today. We’ve come as far as we have because of the observatories, tools, and discoveries that have already occurred, and our knowledge of the laws of physics that helps us interpret them and place them in their proper context. On the other hand, there’s a lot to be excited about as far as new technological developments and observational capabilities on the very near-term horizon. This is a big deal; we’re at the frontiers of our everlasting quest to understand the Universe around us!
That’s why I’m excited to be live-blogging a talk on The Invisible Universe by PhD astronomer and Yale professor Priyamvada Natarajan. One of the top observational cosmologists today, she has a recent book out called Mapping the Heavens: The Radical Scientific Ideas that Reveal the Cosmos. Her talk, available to the public, occurs at 7 PM ET/4 PM PT on March 3, 2021, courtesy of Perimeter Institute.
Tune in then and follow along starting at 3:50 PT (all times to follow in Pacific Time) then, where I’ll be live-blogging the talk from a theoretical cosmologist’s perspective!!
3:50 PM: It’s hard to imagine that only 100 years ago, we didn’t even know what “the Universe” was. The objects we knew of were only a few hundred, maybe some were a few thousand, light-years away. Stars, star clusters, globular clusters, nebulae, etc. Some people argued that the spiral nebulae (and maybe some elliptical ones) were actually entire galaxies unto themselves, far outside the Milky Way, but that was a minority viewpoint. The “great debate” of 1920, which was designed to settle the issue, did no such thing. In fact, the debate moderators gave more points to the “these nebulae are objects within our own galaxy” side, disfavoring the “these are outside teh galaxy” solution.
3:54 PM: It’s such a challenge when you have observations that, well, just aren’t true. A famous paper just a few years before claimed to see stars in a nearby “spiral nebula,” the Pinwheel Galaxy (Messier 101), moving over time: rotating with the object. If this were a galaxy, far outside of the Milky Way, these stars would be moving much faster than light. Therefore, the argument went, this object must be close by, and within our galaxy.
3:57 PM: But when we look at the Pinwheel in detail, even 105 years after those observations claiming rotation, we see that no such thing has occurred. The only objects that have moved at all, in fact, within this field of view, are the rare intervening star that’s present within our own galaxy along the line-of-sight. This object is a galaxy, it is rotating, but it takes hundreds of millions of years to complete a revolution; we cannot detect the motions of the stars in this galaxy: more than 10 million light-years away.
3:59 PM: The lesson? We have to not only measure something occurring to conclude that it’s real and true, we have to both:
- measure it to a certain level of statistical significance,
- and we have to account for our systematic errors and uncertainties.
Generally, the way to do this is to demand a level of quantitative rigor that was missing from the earlier studies, and also to demand repeatability and independent confirmation, something that not only couldn’t be obtained for those rotation results, but that was hotly disputed by many in the field.
In short: if a new effect is real, there should be multiple independent ways to verify it, or at least multiple independent teams working on detecting it without the influence of the other.
4:00 PM: And, here we go! It’s very exciting to have a public lecture series still going — an event for the general public — during the current global pandemic. Glad that Perimeter Institute was able to make this work!
4:04 PM: I’m very curious to see how the slides work: can we see both the speaker and the slides simultaneously?
4:06 PM: Nope. We can see Priya’s slides and hear her voice. Still, it gives us something to focus on, and I’m hopeful this will still be an engaging and dynamic format. Let’s go!
4:09 PM: Let’s be clear about something: the evidence for supermassive black holes was pretty overwhelming much more than 10 years ago. The high-intensity radiation, seen particularly in the radio (lower left) and X-ray (lower right), must be originating from a very massive, energetic engine that itself emits no light. In addition, we had been observing stars orbiting the galactic center since the late 1990s, with again, no light emitted and evidence for an object being there of millions of solar masses quite robustly.
There’s a lot more that we’ve done since, but the idea that these central objects were anything other than a black hole weren’t really taken seriously.
4:12 PM: I thought it was worth pointing out, when we look at the geocentric vs. heliocentric models, that both models could explain what was observed. It was only long after Copernicus, with the advent of Kepler’s idea of elliptical orbits, that the data was actually fit significantly better by the heliocentric model than via any other model.
4:15 PM: Priya mentions, but doesn’t belabor (and I think it’s worth belaboring!), the multiple independent lines of evidence for dark matter. We have a whole slew of observations we can make, and I hope she goes through them. But if you want to be quantitative and ask, “how much of the Universe’s energy is in the form of black holes,” you get an answer on the order of ~0.001% of the Universe’s total energy. What’s also remarkable is that this is almost exactly equal to the amount of negative gravitational potential energy that came from the collapse of the matter that formed the black holes themselves!
4:18 PM: What Priya is talking about is something you can see in the above graph: three different simulations with three different types/abundances of dark matter. If the Universe is too clumpy or not clumpy enough, or clumps differently on various scales than our simulations predict, we would certainly be able to rule out those scenarios. The only way we can get the large-scale structure of the Universe to match observations is with the addition of dark matter.
4:21 PM: Okay, this is worth showing. Do you see this graph? It shows, based on the observed redshift, how fast those individual galaxies within the Coma Cluster are moving relative to our line-of-sight. Note that the “slowest” galaxies move away from us at about ~4700 km/s, while the “fastest” move at ~8900 km/s. The difference of ~4200 km/s is enormous, indicating that there must be enough mass present to keep these galaxies all bound together, even with these very high speeds.
Although many disputed this — not the observations, but the interpretation, claiming that there could be “dark normal matter” explaining everything — this type of observation is now a vital piece of evidence in understanding the dark matter puzzle.
4:24 PM: I want you to appreciate the difference between a galaxy with normal matter only, which would rotate like the galaxy shown on the left does, with the one on the right, which assumes a dark matter halo. If this were the only piece of evidence we had, I freely admit, the dark matter explanation wouldn’t be nearly as compelling as it is with a view to the full suite of what’s out there.
4:27 PM: Okay, Priya is showing a diagram of strong gravitational lensing right now, and that is a very important part of the puzzle. As she shows, when you have a large mass that intervenes between a distant light source, the proper configuration can cause it to act like a strong lens, which can produce highly magnified images, multiple images, and distorted images.
But what’s much more powerful is weak gravitational lensing, and that’s far more general. What happens is that galaxies are normally randomly oriented: the bottom left panel, above, is what they should naturally look like. However, where you have a large mass — a galaxy cluster, for instance — intervening, you see these distortions in shape and orientation of these galaxies. If you do a statistical analysis, you’ll find that you can actually infer the mass and the mass distribution of the foreground clusters. Here’s a brilliant image showing the mass reconstruction, from exactly this type of lensing, for a galaxy cluster. This was an early example, from 1998.
4:31 PM: The nice thing about gravitational lensing is that for every foreground mass we’ve ever observed, there always are background light sources. The more sources there are, and the better we measure them, the more and better the mass reconstruction of the foreground object will be. For the richest galaxy clusters of all, this results in the greatest amounts of gravitational lensing. This enables us, among other things, to observe galaxies that would otherwise be too distant and too faint to be seen with current equipment.
4:34 PM: So, you want to see some excellent examples of strong gravitational lensing? Priya chose to show you Abell 2218, which has some pretty prominent features, for sure. But did you know that there are many enormous, massive, distant galaxy clusters not only throughout the Universe, but in the Abell catalogue?
Check out some of my favorites!
They include Abell 370:
Abell S1063:
Abell 2667:
and Abell 2744.
4:39 PM: Ha! Priya is showing a plot from a novel paper that I am in the process of writing up for a new article that is slated to publish in about ~6 hours from now. Isn’t life interesting!
DAMA/LIBRA, and I’m speaking freely here, is notoriously an outlier when it comes to dark matter experiments. Yes, we are yet to detect dark matter, and if Priya wanted to be less diplomatic than she was, it would’ve been completely justified.
4:42 PM: OK, we’re clearly at the black hole part of the talk now. I like the idea of thinking about black holes in multiple different ways. The “strength of the pull of gravity” is a good one: if your escape velocity is the speed of light, you can’t escape, and so if you pack enough matter into a small enough volume of space, anything will become a black hole.
4:45 PM: Black holes can also arise from collapsing matter from the deaths of supermassive stars. It isn’t just supernovae, mind you, but also there are other mechanisms, such as direct collapse, that can cause them, too.
This isn’t just theoretical; we’ve literally seen very massive stars just “disappear” with no supernova explosion! They must have become black holes.
4:48 PM: Are black holes really a “puncture” in spacetime? Believe it or not, this is an equally valid way to look at black holes, and is actually quite general.
One of the fun things is that Schwarzschild (massive but non-rotating) black holes do indeed behave as punctures, where you literally have a “hole” (or, mathematically, a topological defect) in spacetime itself: a discontinuity. In a Kerr (rotating and massive) black hole, which is more realistic, black holes aren’t quite “holes” anymore, but rather entities that actually lead… well, weren’t not exactly sure where, but the answer appears to be “somewhere” rather than nowhere, or to a point-like singularity. Kerr black holes have ring-like singularities, and unlike in Schwarzschild black holes, you can never even reach them!
4:50 PM: I do have to say, this new format took a little bit of getting used to, but I’m finding myself just as absorbed into Priya’s talk as I have been at any previous Perimeter Institute public lecture. That’s a win for technological solution to modern problems!
4:54 PM: Now, Priya is talking about supermassive black holes, and there’s an enormous question surrounding them: how do they form and grow in our Universe?
We know that they feed; we know where they live; and we know how they affect their environments. But there are many, many open questions, with some groups actively debating whether when galaxies merge, whether the supermassive black holes are likely to merge (or not) within the present age of the Universe. If not, we might find a large number of binary (or more) supermassive black holes at the centers of highly evolved galaxies!
4:57 PM: Intermediate mass black holes should exist, but they may not be very common. The place we’d been looking for them has been largely within globular clusters: collections of a few hundred thousand stars, but those detections have been disputed and few in number. But the way we successfully detected them has been, as Priya alludes to, is when a star passes close to one of these intermediate mass black holes, tearing it apart.
These tidal disruption events are extremely energetic, transient phenomena, but the advent of automated most-of-the-sky telescopes, like the Zwicky Transient Facility or Pan-STARRS, has given us a virtual explosion of these objects over the past few years!
5:01 PM: And, of course, there are ripples in spacetime produced by merging black holes, even of the supermassive variety. What Priya maybe alluded to but didn’t show is there’s currently a puzzle with this scenario: the two original supermassive black holes will eject or swallow the entirety of the gas in the surrounding environment before the black holes get close enough that gravitational radiation will bring them into each other.
5:03 PM: Here is the animation Priya loves so much: the ripples from a gravitational wave merger, that shows how spacetime contracts and rarefies in mututally perpendicular directions as a gravitational wave passes through it.
5:05 PM: Okay! This is what I came for: Priya is talking about her research, specifically about how we get black holes that are massive enough early enough to grow into what we know today as the earliest supermassive black holes in the young Universe.
Here are some of the earliest, if you’re curious.
5:08 PM: Priya is now showing an animation of when you expect black holes of a certain mass to arise in the Universe. Note that these predictions do not match up with what we see; what we see at early times are too massive!
5:11 PM: This was a good talk! Way to go, Priya, and it covered a lot of ground in very excellent depth. I liked how accessible it was, but also what a good job she did of bringing everyone up to speed on where the modern frontiers are. The only thing I wish is that she saved more time for talking about how we’re going to address the issues at the frontiers, beyond saying, “James Webb Space Telescope.”
But also, I do love the James Webb Space Telescope.
5:13 PM: I like how appropriately open-minded Priya is about dark matter. Here’s what we think it is, but here’s also the limits to how far we’ve tested it, and how robust and successful are the alternatives? We question, but we subject our questions to the appropriate level of scrutiny.
5:15 PM: Who said that?! Who said, “we’ll know what dark matter is in the next ~10 years,” without qualifying that with the necessary, “if we’re lucky?” Priya is talking about WIMPs and axions, which are fashionable, with all possible incarnations of dark matter, which are near-infinite, and those are not the same.
We are looking where we can look, and that’s a very smart and valuable effort. But if it’s “none of the above,” that won’t necessarily cause a rethink of the particle nature of dark matter. We doubt, and we try to verify, but we don’t know what nature is doing. We can only measure what we can measure, and draw provisional conclusions based on what we do (and don’t) see.
5:18 PM: Fun question: what will we think is a “quaint” idea 100 years from now that’s fashionable today? Priya says, “multiverse,” but she’s also correct: it can’t be empirically substantiated. (Probably.) She also says that our mind imposes limits, but maybe those limits aren’t there. Just like Copernicus couldn’t have imagined spacecraft leaving the Solar System, who knows what we can’t imagine!
5:23 PM: Final question: what’s the most important trait for a successful physics career? She picked two:
- Resilience.
- And the capacity to imagine and dream.
Bam! What a great answer, and a very good talk! Thanks for joining me, and see you back here, well, in just a few hours, when I’ll tell you the story of how the world’s most controversial dark matter experiment has just been, err, handed their hat.
Starts With A Bang is written by Ethan Siegel, Ph.D., author of Beyond The Galaxy, and Treknology: The Science of Star Trek from Tricorders to Warp Drive.