Ask Ethan: Do any particles not have antiparticles?
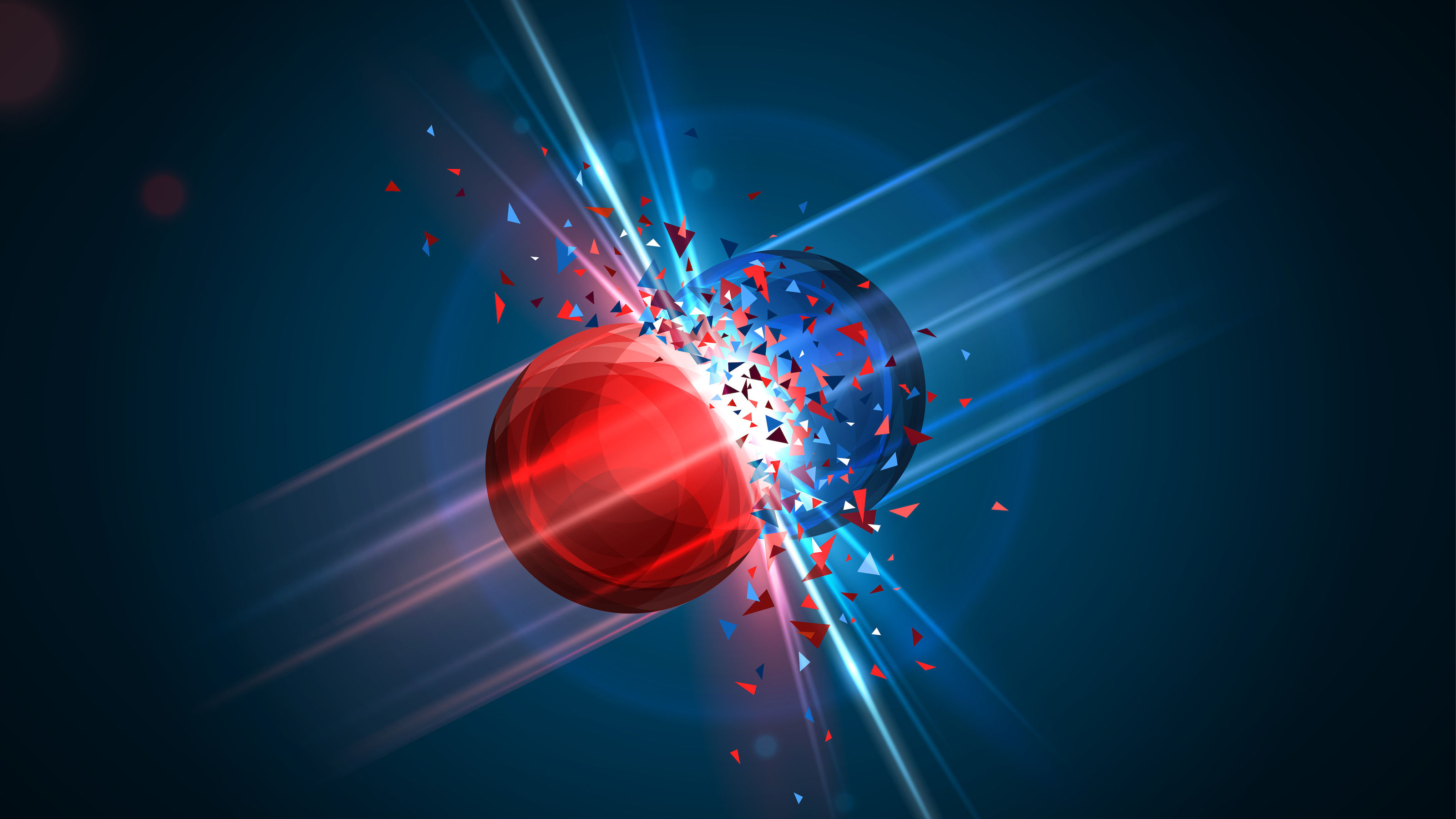
- Here on Earth, everything is made of matter particles: atomic nuclei are made of protons and neutrons, and are orbited by electrons, with these systems binding together to make molecules, ions, and more.
- At very high energies, we can also create antimatter, which is made of antiparticles. When matter and antimatter of equal-and-opposite types meet, they annihilate away.
- But if we go all the way down to an elementary level, and take a full census of all the fundamental entities, we find that not every particle has an antiparticle counterpart. Here's what that means.
Here in this Universe, there are certain laws of physics that never appear to be broken. No information-carrying signal, for instance, can ever move faster than the speed of light. Energy, if you account for all of the different types that exist, can never be created or destroyed: only conserved. Electric charge, linear momentum, and angular momentum are all similarly conserved. And, to the best of our knowledge, the only way to create new matter particles is to create an equal number of new antimatter particles, as we’ve never observed a single reaction that has either created or destroyed a net amount of matter over antimatter, or the other way around.
But are all of the entities in our Universe either “matter” or “antimatter” in some sense, or are there particles out there that don’t have antiparticles at all? That’s the question of David Wiser, who wants to know:
“I was wondering if there are any elementary particles that do not have corresponding antiparticles? The only two that seem to fit this category are the photon and graviton. Are there others? Is there any significance to not having an antiparticle? Is this related to their traveling at the speed of light?”
There’s a lot to unpack here, but the short answer is yes: not every elementary particle has a corresponding, distinct antiparticle. The long answer is even more interesting. Let’s dive in and find out!
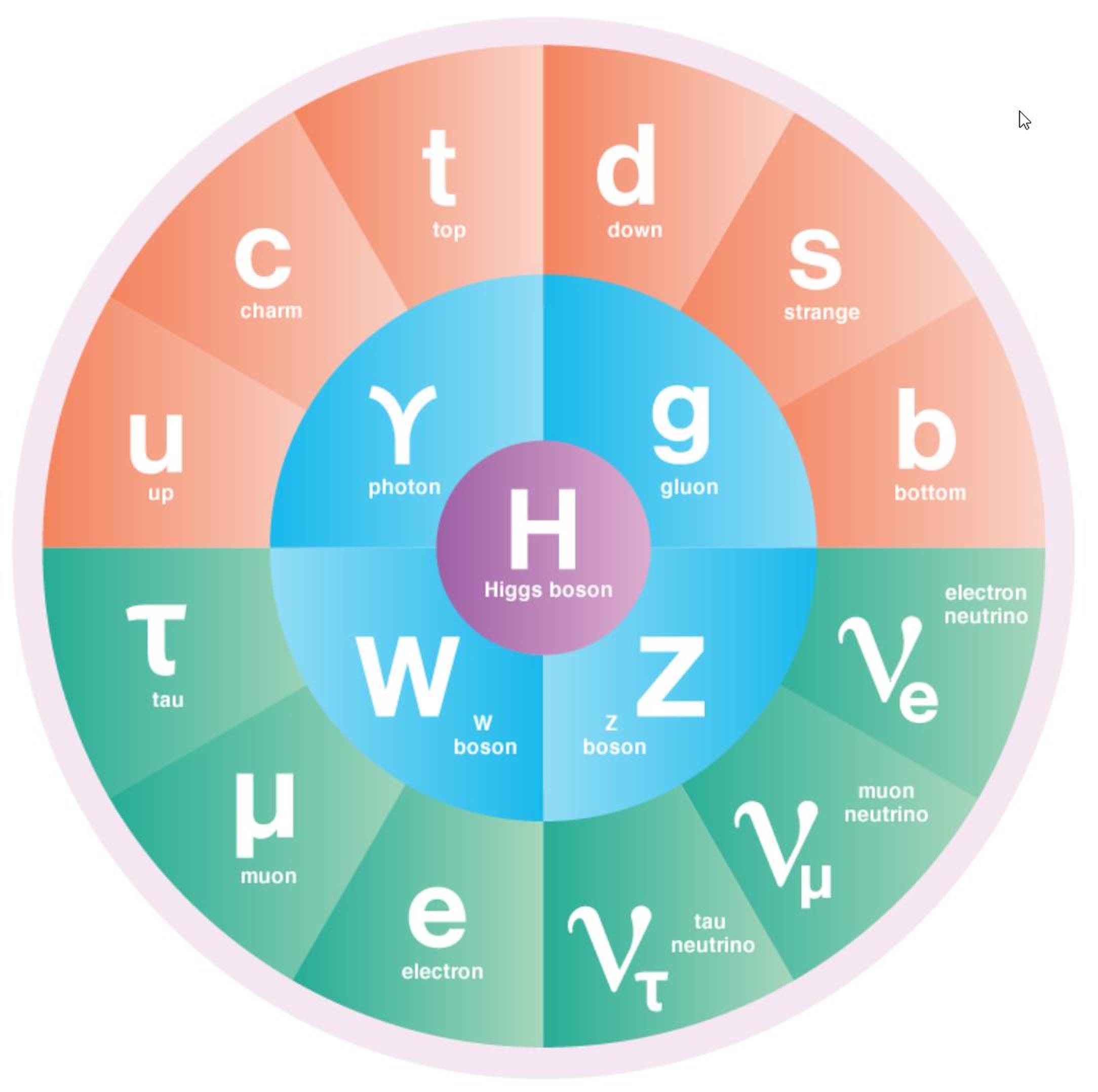
Above, you can see the particles of the Standard Model. These represent all of the presently-known and discovered fundamental particles that make up the Universe, and they still don’t account for two of the greatest mysteries in all of physics: dark matter and dark energy. The particles within the Standard Model come in a few different varieties:
- there are quarks, which have masses, color charges, electric charges, spins, and come in six flavors (up, down, strange, charm, bottom, and top),
- there are the charged leptons, which have masses, electric charges and spins, and come in three different families (electron, muon, and tau),
- there are the neutral leptons, or neutrinos, which have masses and spins, but the “flavors” that you can observe them to have (electron, muon, and tau) are different from the masses (1, 2, and 3) that you can observe them to possess,
- and then there are the force-carrying particles: the gluons (of which there are 8, that carry the strong nuclear force), the W-and-Z bosons (of which there are 3, the W+, W–, and Z0, that carry the weak force), and the photon (of which there’s only one, and that carries the electromagnetic force),
- plus the Higgs boson,
- and if we’re being generous and we assume that gravity is an inherently quantum force (which it may not be), then there should also be the graviton to carry the gravitational force.
That’s a lot of particles, but there are a large number of “antiparticles” that we don’t normally talk about as well, so I’ve created my own Standard Model chart for you, below, that better illustrates this.
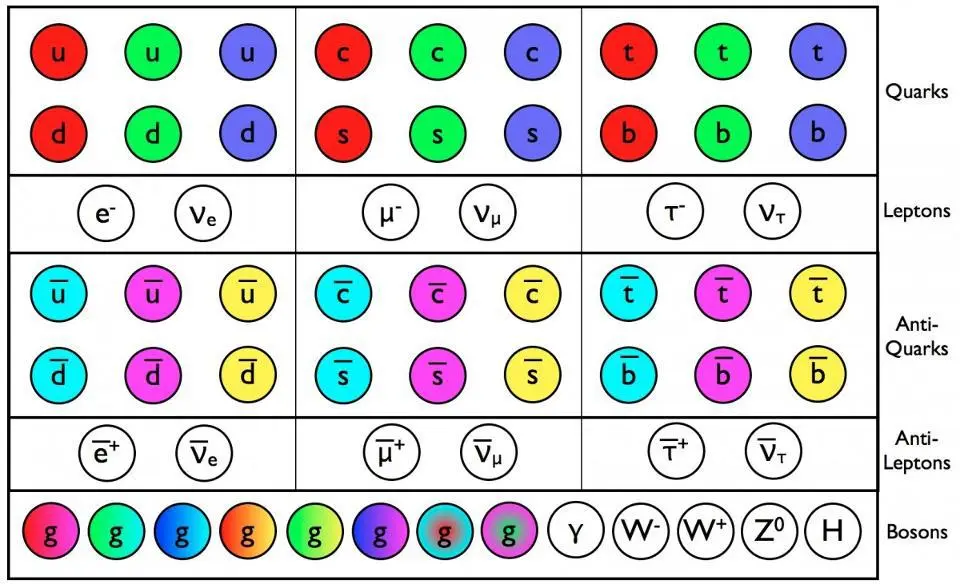
You can see very clearly that, for the first three classes of particle — the quarks, the charged leptons, and the neutral leptons/neutrinos — there is indeed a corresponding antiparticle for each individual particle species.
- Each antiquark has the same mass, the same possibility for spins (+½ and -½), but opposite electric charges and opposite color charges compared to its quark counterpart. In addition, because it takes three quarks to make up a baryon, each quark has a baryon number of +⅓, while each antiquark has a baryon number of -⅓.
- Each charged antilepton (the positron, antimuon, and antitau) has the same mass, the same possibility for spins (+½ and -½), but opposite electric charges (+1 for antileptons, instead of -1 for leptons) and opposite lepton number (-1 for antileptons, as opposed to +1 for leptons) from their particle counterparts.
- And then each neutral antilepton, or antineutrino, has the same set of mass eigenstates (1, 2, and 3) as their neutrino counterparts, has the opposite spin (all neutrinos are left-handed, with spin -½, while all antineutrinos are right-handed, with spin +½), and again the opposite lepton number (-1 for antileptons, as opposed to +1 for leptons) from their neutral lepton/neutrino counterparts.

That’s because the quarks and leptons are a special class of particle known as a fermion: a particle with half-integer spin (e.g., ±½, or ±1½, or ±2½, etc.) inherent to it. Fermions are matter particles, and their antiparticle counterparts, the antifermions (which include antiquarks and antileptons), are antimatter particles.
If you consider the Earth and everything on it, it’s all made out of fermions. Every atom is made out of protons, neutrons, and electrons, where an electron is a lepton (a fermion), protons and neutrons are each composed of three quarks (three fermions), and where each atom has a positive baryon number (the number of quarks, divided by three) and a positive lepton number (the number of electrons).
If you wanted to, you could flip the script and imagine an antimatter version of Earth or anything on it: made of anti-atoms. Anti-atoms would be made out of antiprotons, antineutrons, and positrons, where antiprotons and antineutrons are fundamentally composed of three antiquarks (antifermions) each, carrying negative baryon number (because they’re antibaryons), and where positrons, the antimatter counterpart of electrons, carry negative lepton number to them.
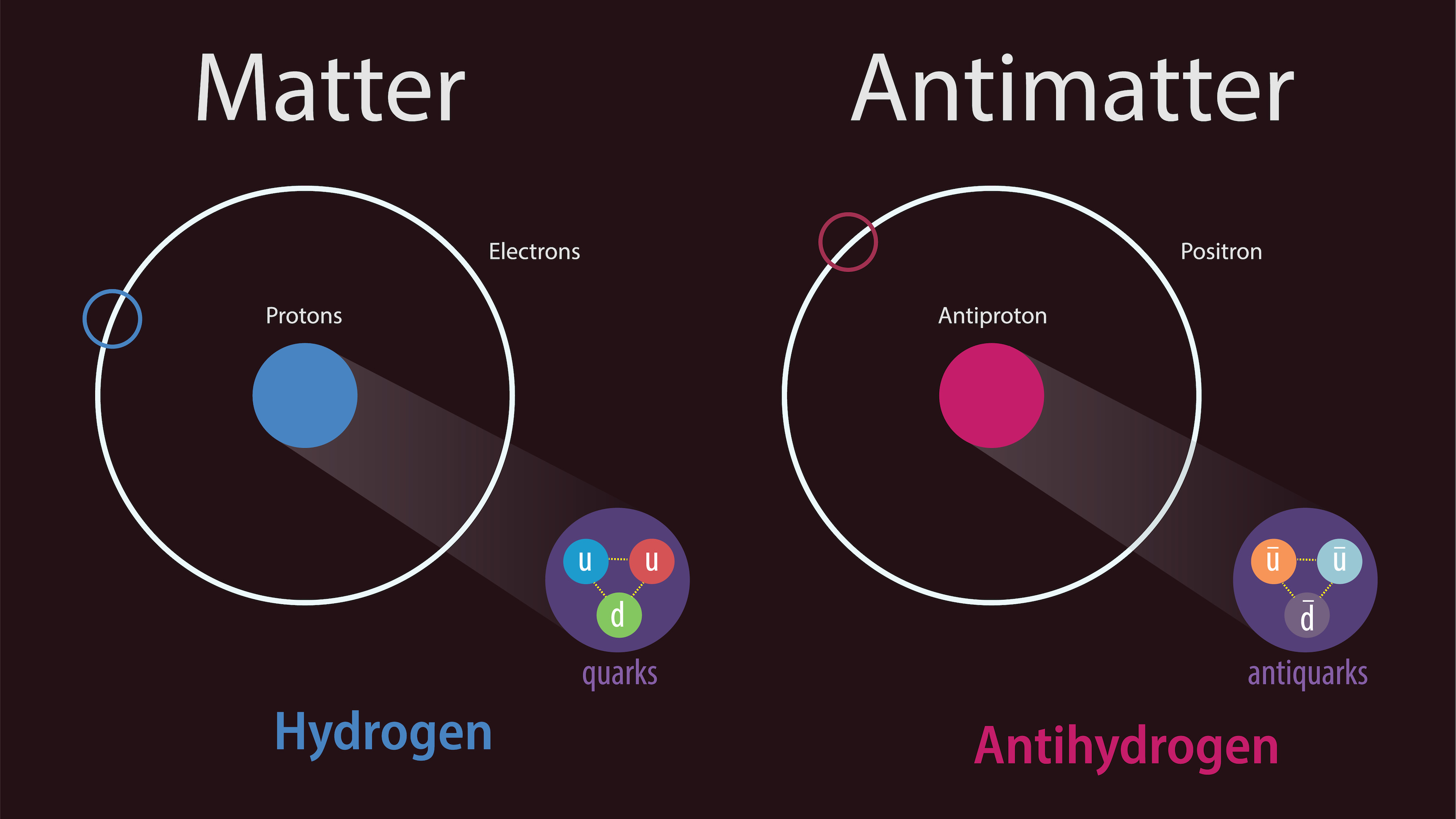
When we talk about “matter” and “antimatter,” we’re always talking about the fermions (or antifermions): things made out of quarks and leptons (or antiquarks and antileptons), and that carry either baryon number or lepton number, whether positive or negative, or both.
But that very clearly doesn’t account for all of the particles (and antiparticles) that are present within the Standard Model. After all, we still have:
- the 8 types of gluon, which mediate the strong nuclear force,
- the 3 types of weak boson, the W-and-Z bosons, which mediate the weak nuclear force,
- the photon, which mediates the electromagnetic force,
- and the Higgs boson,
- plus, depending on how confident the physicist you’re talking to is about gravity being an inherently quantum force, possibly the graviton as well.
Unlike the fermions we were talking about earlier, however, absolutely none of these particles can be considered as either “matter” or “antimatter,” as they all possess neither baryon number nor lepton number. These aren’t fermions at all, but rather are examples of bosons: particles with integer (0, ±1, ±2, etc.) spins.
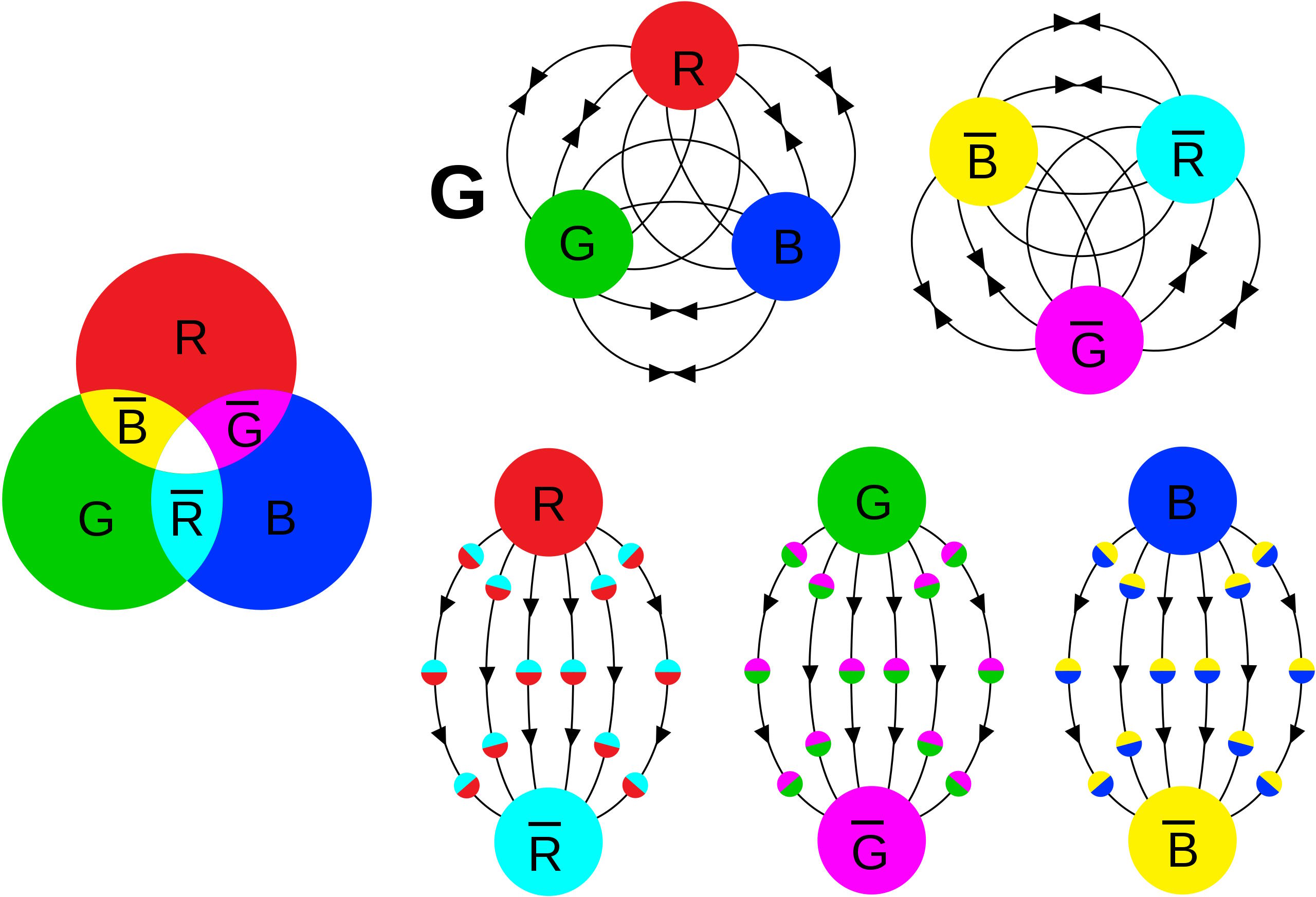
Bosons are interesting, because they’re neither inherently members of either the matter or antimatter families, but interact with not only fermions (matter) and antifermions (antimatter), but also themselves.
Take the quarks, for example. If you put three of them together, you can make a baryon, and it’s the force-carrying gluons that hold the baryons together. Alternatively, you can take three antiquarks and put them together to make an antibaryon, and still, it’s the same eight force-carrying gluons that hold those antibaryons together. And if you instead bind a quark to an antiquark, you’ll make a short-lived unstable particle known as a meson, and yet again, it’s the very same gluons that hold mesons together.
If you then go ahead and ask the question of what the gluon’s antiparticles are, you’ll find out a fact that might seem surprising at first: it’s the other gluons! Whereas a quark has a color inherent to it (red, green, blue) and an antiquark has an anticolor inherent to it (cyan, magenta, yellow), gluons are made of color-anticolor combinations. The red-magenta gluon is the antiparticle to the green-cyan gluon; the red-yellow gluon is the antiparticle to the blue-cyan gluon; the blue-magenta gluon is the antiparticle to green-yellow gluon. The gluon is the antiparticle to the gluon, but no gluon is more “matter-y” than “antimatter-y” in any sense; they’re a separate type of particle.

There’s a similar story when it comes to the weak force. Imagine that you have a neutron: a matter particle made of two down quarks and one up quark. Neutrons, unless they’re bound together into a stable atomic nucleus, are inherently unstable particles: capable of decaying into a proton, an electron, and an electron antineutrino. (Note how this decay still conserves baryon number, lepton number, and electric charge!) The way this decay takes place is by the following method:
- the down quark emits a (virtual) W– boson,
- transforming it from a down quark into an up quark (and hence, the composite particle from a neutron into a proton),
- and then the (virtual) W– boson decays into an electron and an electron antineutrino,
conserving everything that particle physics demands: energy, momentum, electric charge, spin, color charge, etc. We also conserve lepton number and baryon number, as the W– boson couples to both fermions and antifermions.
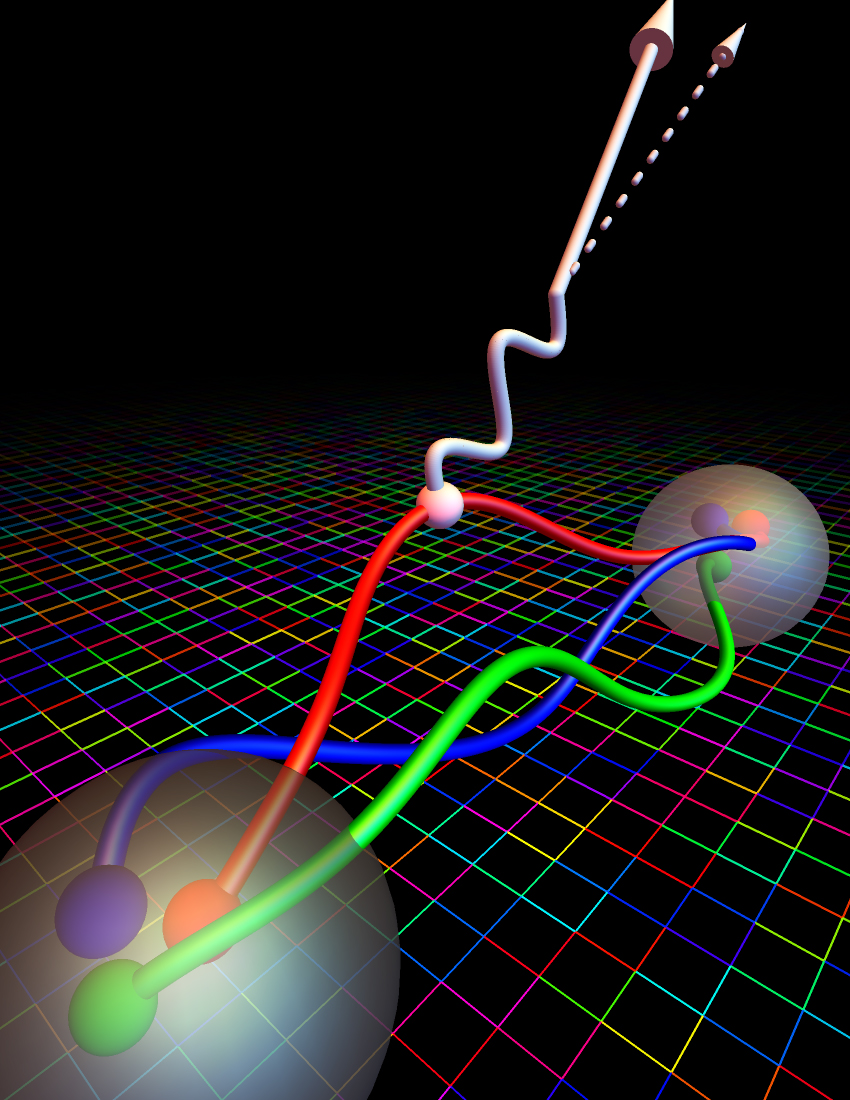
We can consider the antimatter counterpart of this reaction now: what happens when you have a free antineutron and it radioactively decays? Antineutrons are made of three antiquarks: one anti-up and two anti-downs, and when they radioactively decay, one of the anti-downs decays into an anti-up, plus a positron and an electron neutrino. The way this decay takes place is via the following pathway:
- one of the antiquarks, an anti-down one, emits a (virtual) W+ boson,
- transforming it from an anti-down into an anti-up (and hence, the composite particle from an antineutron into an antiproton),
- and then the (virtual) W+ boson decays into a positively charged positron and a neutral electron neutrino,
again conserving all of the same necessary quantities in these interactions, including lepton number and baryon number. Even though the W– and W+ particles are neither “matter” nor “antimatter,” they are one another’s antiparticles: if you were to collide them, they’d annihilate, and can produce any particle-antiparticle pair admissible by the laws of energy conservation: from Einstein’s E = mc².

Sometimes, the antiparticle of a boson is a different boson, as in the examples of six of the gluons (mediating the strong force) and the W– and W+ particles that are weak force mediators. But that still leaves us with a few other particles that we haven’t addressed yet:
- the photon,
- the Z0 boson,
- the Higgs boson,
- the two gluons that are inherently equal mixes of color-anticolor combinations,
- and the graviton.
Just as you’d suspect, because these are all bosons, they’re neither inherently matter nor antimatter, but some state that couples to both but is inherently neither.
But as you might not suspect, in each of these cases, each of these bosons is its own antiparticle! If you collide a:
- photon with a photon,
- a Z0 boson with a Z0 boson,
- a Higgs with a Higgs,
- the equal-mixed gluons with the same species of gluon,
- or a graviton with a graviton,
you’ll get the same annihilation phenomenon you’d get from colliding matter with antimatter: where the initial two quanta disappear, and in their place, any particle-antiparticle pair (including the above mentioned particles that are their own antiparticles) can be created. So long as you obey the necessary conservation laws, you can make anything up to the amount of energy you have available: via Einstein’s E = mc².

So to answer the original question: every known particle has an antiparticle. When that particle and antiparticle collide, they annihilate away and can create any new particle-antiparticle pairs that are allowable by laws of nature and the energy of the annihilating collision. Sometimes, the particles are fermionic matter, and in those cases, the antiparticles are antimatter. Sometimes, the particles are bosonic, in which case both those particles and their antiparticles are neither matter nor antimatter.
For some of the bosonic cases, such as for some of the gluons and both of the charged weak bosons, particles are distinct from their antiparticles, as two different species must collide in order to annihilate. For other members of the boson class, particles behave as their own antiparticles, and this should include two of the gluons, the neutral weak boson, the photon, the Higgs boson, and the graviton. It’s not quite correct to say that “these particles have no antiparticle counterpart,” but rather more correct to state that these particles are their own antiparticle.
Note that all fermions are massive, whereas some of the bosons are allowed to be massless, but that whether a species is massive or massless bears no relation to whether it’s allowed to be its own antiparticle or not. And remember: these are only for the known particle species contained within the Standard Model. By the time we get to the bottom of the dark matter puzzle, we may yet discover something novel and unanticipated about nature. In the end, after all, we can only be certain about what can be observed and measured, and until we get there, we will have no choice but to continue the search.
Send in your Ask Ethan questions to startswithabang at gmail dot com!