No, Today’s Stars Are Not The Same As Yesterday’s Stars
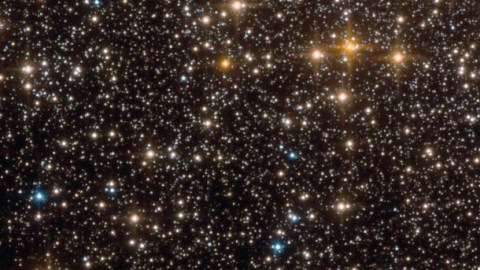
The Universe’s idea of a ‘typical star’ has changed dramatically over time.
When you look out at the Universe today, you’re not seeing it exactly as it is at one particular instant in time: now. Because of the fact that time is relative and light isn’t instantaneously fast — it can only move at the large, but not infinite, speed of light — we’re seeing things as they were when they emitted the light that only now is arriving. For an object like our Sun, the difference is cosmically minuscule: the Sun’s light arrives after a somewhat paltry journey of only 150 million km (93 million miles), which takes just a little over 8 minutes to complete.
But for the stars, star clusters, nebula, and galaxies we see across the Universe, because of their great cosmic distances, we’re seeing them as they were a much longer time ago. The closest stars are only a few light-years away, but for the objects that are millions or even billions of light-years distant, we’re seeing them as they were a significant fraction of the Universe’s history ago. The light that we receive from the most distant galaxy discovered so far — GN-z11 — was emitted when the Universe was just 407 million years old: 3% of its current age.
With NASA’s James Webb Space Telescope launching later this year, we’re poised to go back even farther. The stars from back then are fundamentally different from the stars we have today, and we’re about to find out exactly how.
The stars that exist today, for the most part, fall into two categories.
- There are stars similar to our Sun: with lots of elements other than hydrogen and helium in them, that were formed many billions of years after the Big Bang, and include lots of materials that must have been formed in previous generations of stars.
- There are stars that are fundamentally less evolved than our Sun: formed much closer back in time to the Big Bang than our own, with only a small amount of elements other than hydrogen and helium, whose material only includes a small amount that went through prior generations of stars.
While that first type of star — what astronomers call “metal-rich” stars, since to an astronomer, any element on the periodic table that isn’t hydrogen or helium counts as a metal — can come in all different sizes, masses, and colors, the same isn’t true for that second type of star. The “metal-poor” stars in our Universe are overwhelmingly small, low in mass, and red in color.
Why are the metal-rich stars so diverse, but the metal-poor stars are all so similar to one another? The answer is simple: the metal-rich stars come in a wide variety of ages, but the metal-poor stars are all very, very old.
When we look out at the Universe and ask the questions, “where does it form stars,” we get a lot of different answers. You can have very small, isolated clouds of gas that cool and contract, eventually forming only a small number of stars. You can have larger clouds of gas that fragment into smaller clumps, producing a substantial cluster of stars in one location but only a small number elsewhere. Or you can have very large clouds of gas leading to intense periods of star formation, where thousands, hundreds of thousands, or even millions upon millions of stars are formed all at once.
Overwhelmingly, though, the majority of stars in the Universe are created during these major events of star-formation. It’s a little bit like the reverse of HBO’s Game of Thrones TV show: you might go for a few episodes where no one dies or only a few casualties occur here or there, but then there are these incredibly violent episodes where large numbers of people all die in one location. Well, star-formation is a bit like the opposite of that: it’s mostly quiet and steady, with a new star here or there, but the overwhelming majority of star-formation occurs in these bursts that create enormous numbers of new stars all at once, of all different varieties.
Today, whenever you make a large number of new stars all at once, here’s what happens.
- The largest, most overdense regions of matter start to contract the fastest; gravitation is a game of runaway growth, and whichever regions have the greatest amounts of mass collapse the earliest.
- The contracting matter has to cool, radiating away the energy that’s gained from this gravitational contraction.
- The richer in (astronomical) metals the gas is, the more efficient it is at radiating heat away, meaning that it’s easy for the gas to collapse and form new stars.
- And how easy or hard it is for gas to collapse and form new stars determines what astronomers know as the “initial mass function,” which tells us what types, masses, colors, temperatures, and lifetimes of the stars that form will be.
Whenever you have a large star-forming region in the modern Universe, to the best of our knowledge, you always wind up with roughly the same sets of stars inside.
On average, the mass of a typical star will be about 40% the mass of the Sun. Stars that are lower in mass than our Sun are going to be redder in color, less luminous in their intrinsic brightness, lower in temperature, and longer-lived (because the lower rate of fusion that occurs) relative to us. However, the overwhelming majority of the stars that are formed, somewhere around ~80% of them, will be even less massive than the average star.
That leaves a lot of room for some very massive stars to form. About 15% of the stars that form will still be lower in mass than our Sun, but more massive than that ~40% figure, leaving only 5% of all stars (by number) that are more massive than our Sun. But those stars are predominantly brighter, bluer, hotter, and also shorter-lived than our Sun is. The largest collection of them that we know about are found in a massive star-forming region in the Tarantula Nebula. Despite being located in the Large Magellanic Cloud, only the fourth largest galaxy in our Local Group, it’s the largest star-forming region around for almost 10 million light-years.
Even though the stars inside look like they’re predominantly blue and bright, this isn’t exactly the case. Instead, the stars that are bluest and brightest are the stars that are the most prominent and easily seen. The stars inside the Tarantula Nebula are already some ~165,000 light-years away, and so it’s only the brightest ones that pop out as clearly visible to us. (It’s worth remembering that the closest star to our Sun, Proxima Centauri, was only discovered about 100 years ago. Even today, knowing exactly where it is, it takes a telescope about the diameter of your outstretched hand to see it at all.)
About 20% of the stars inside the Tarantula Nebula, like in any region that’s recently formed stars, are between about 40% and 800% the mass of our Sun. They will, typically, live for hundreds of millions to a few billion years, burn through the hydrogen in their cores, swell into red giants, fuse helium into carbon, and then expel their outer layers while their cores contract into white dwarfs. This process of stellar death forms what we call a planetary nebula, and is primarily responsible for the origin of many elements, like carbon and oxygen, that are essential to the biology and chemistry found on Earth.
At the center of the Tarantula Nebula, however are the most massive individual stars we know of, with dozens of stars exceeding 50 solar masses, two heaping handfuls of stars over 100 solar masses, and the most massive one of all, R136a1, reaching an estimated mass of 260 Suns. The bright, blue stars burn through their fuel incredibly fast, shining many millions of times brighter than our own Sun. They also live for incredibly short timespans, burning through their core’s fuel in as little as 1-to-2 million years: one ten-thousandth the lifetime of a Sun-like star.
The stars that are more massive than about 8 solar masses, when they’re born, will eventually end their lives in a core-collapse supernova, which recycles the heavy elements that were forged inside the star — both during its life and during the supernova process — back into the interstellar medium, where it enriches the material that will be used for future generations of stars.
This recycled material from supernovae is primarily responsible for the origin of a few dozen of the elements found in our Universe, but there are other ways that these stars contribute. In addition, the remnant at the core will be either a black hole or a neutron star, and both of those play a role in populating our Universe with the elements of the periodic table.
Neutron star mergers provide the majority of many of the heaviest elements in the Universe, including gold, platinum, tungsten, and even uranium. While our Sun might be a “singlet” star, don’t be fooled: about 50% of all stars exist in multi-star systems with two or more stars inside, and if two massive stars both become neutron stars, a merger is all but inevitable.
Meanwhile, black holes and neutron stars accelerate matter around them, creating high-energy particles known as cosmic rays. These cosmic rays collide with all sorts of particles, including some of the heavy elements that were created in earlier generations of stars. Through a cosmic process called spallation, where cosmic rays blast these heavy nuclei apart, some lighter nuclei are produced, including significant fractions of the lithium, beryllium, and boron (elements 3, 4, and 5) in the Universe.
The thing is, these are the stars that have formed in the already-enriched Universe: the ones that formed recently or are still forming today. Earlier on, there were fewer generations of stars that lived-and-died, and that means that there were fewer heavy elements in the stars that formed long ago. Those metal-poor stars exist in great abundance in the outskirts of our galaxy: members of ancient structures known as globular clusters. But these are already many billions of years old; all the massive stars in them already died long ago.
What are metal-poor stars like when they’re just born? And, going even farther back in time, what was the very first generation of stars like: the ones that were made of elements that only were created in the hot Big Bang?
In theory, they were far worse at “cooling” than today’s star-forming gas is, and so we expect that the earlier stars are:
- larger,
- bluer,
- more luminous,
- more massive,
- and shorter-lived,
compared to stars just forming today. We fully expect, with the James Webb Space Telescope launching later this year, that one of its prime science goals and discoveries will be to find, identify, image, and study these earliest populations of stars. If it succeeds, we might finally come to understand how good our theories of early star-formation are, and uncover just how massive these early, metal-free stars could get.
What’s a certainty, however, is that the stars in the young Universe were significantly different than the stars that are just coming into existence today are. They were made of different materials; the gas that collapsed to form them cooled at different rates; the sizes, mass distributions, luminosities, lifetimes, and even the fates of these stars were likely very different from the stars we have today. Yet right now, we face the ultimate problem when it comes to learning about them: when we look out at the Universe around us, today, all we see are the survivors.
If we want to find the stars that once dominated the Universe, we have no other option: we have to look extremely far away, to the distant, ancient Universe. Billions upon billions of years ago, the Universe was filled with large amounts of newly formed, massive metal-poor stars, and at even earlier times, the first stars of all. With the advent of the James Webb Space Telescope, we fully expect these elusive stellar populations to not only be revealed to us, but revealed to us in detail. In the meantime, we can take solace in the fact that we understand how the Big Bang, stars, and stellar remnants gave rise to the elements in our Universe.
If we want to fill in the details we’re currently lacking, we have to look deeper, older, and fainter than ever before. The technology to take us there — NASA’s James Webb Space Telescope — is just months away from launch. If you haven’t understood why astronomers are so excited about this observatory up until now, perhaps “the origin of stars, leading to the origin of us” might help you feel some of that excitement for yourself.
Starts With A Bang is written by Ethan Siegel, Ph.D., author of Beyond The Galaxy, and Treknology: The Science of Star Trek from Tricorders to Warp Drive.