Ask Ethan: How can physicists make neutrino beams?

- Whenever you have a nuclear power plant, neutrinos emerge from it omnidirectionally and at high energies: the very first neutrino detections actually came from a nuclear reactor.
- But for particle physics experiments requiring large numbers of high-energy neutrinos, an entirely different method needs to be used, otherwise the neutrino signal would be far too weak and the background would be far too great.
- Fortunately, experiments like DUNE, where the detector is separated from the neutrino production site by ~1000 kilometers or more, have solved this problem. Here’s how!
The neutrino, of all the fundamental particles presently known to humanity, remains in many ways the most difficult particle of all to detect. For any other particle, once you create it, you can easily detect its presence by building a variety of experimental devices — like pixel detectors, calorimeters, and magnetic fields that bend charged particles — that either detect the passage of those particles directly or detect the tracks left by the original particle’s decay products. Only the neutrino and antineutrino require something extra, as each individual particle needs something like a light-year’s worth of lead to have a 50/50 shot of interacting with it.
So how, then, do particle physics experiments that involve neutrino beams actually work? The upcoming Deep Underground Neutrino Experiment (DUNE) experiment requires one, and yet, it can’t work the way that other particle beams work. That’s what David Yager wants to know, asking:
“How efficient will the DUNE experiment be at directing neutrinos at the far target? […] I live near a nuclear power plant, and I have been told that neutrinos are emerging from there at an incredible rate. Are there actually preferred directions of emergence?”
This is a pretty tricky question, because there are two separate things to unpack: how neutrino beams work, and how nuclear power plants work. Let’s dive into them both!
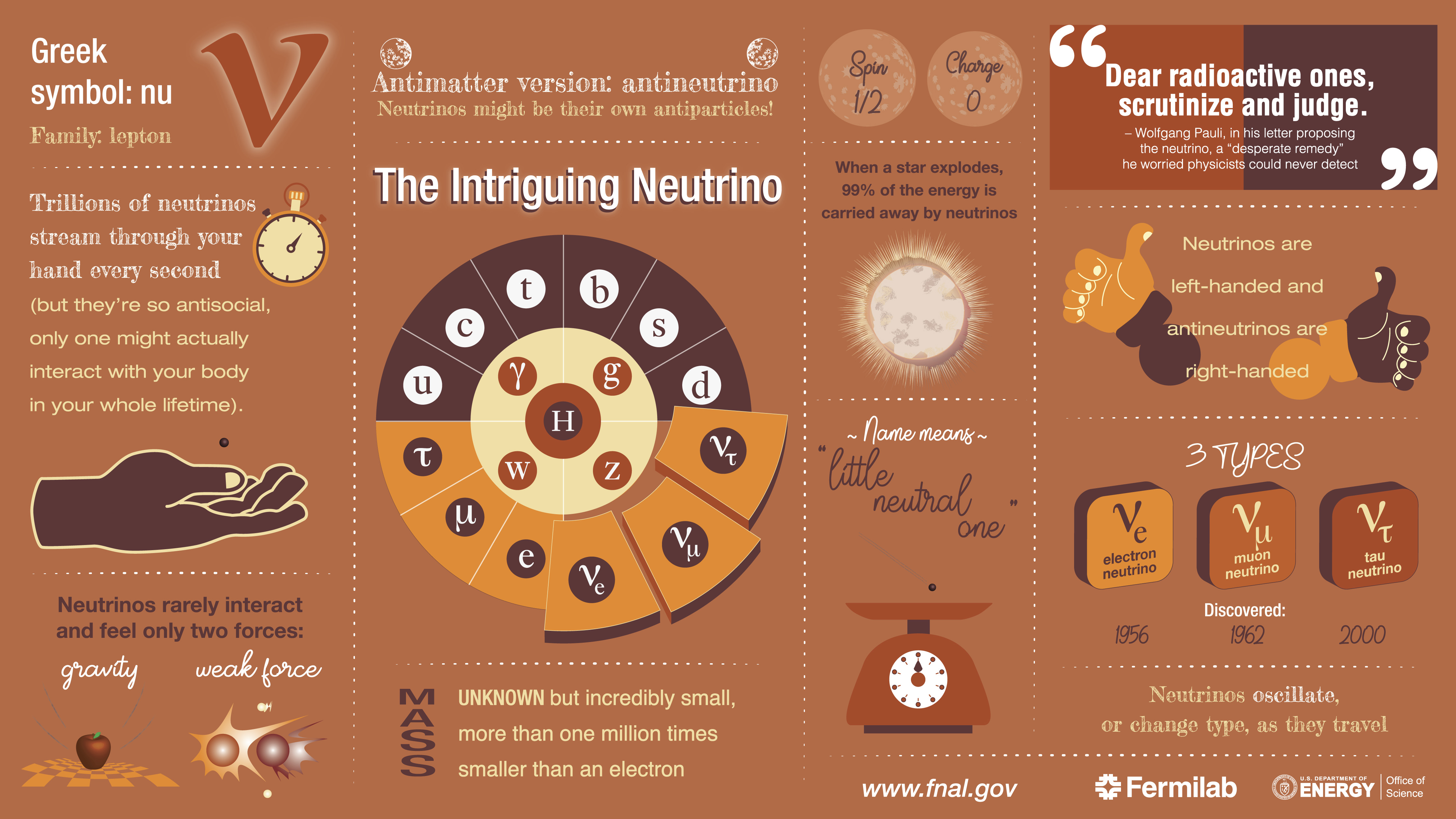
The way you make neutrinos (and antineutrinos), fundamentally, is different than the way you make most particles (and antiparticles) in high-energy physics. Typically, you collide particles together at high energies — electrons and positrons, protons and antiprotons, or even protons with protons — and you create new particles or particle-antiparticle pairs, limited only by the quantum rules that govern the Universe and the amount of energy you have available for particle creation: given by E = mc².
But all the other quarks and leptons in the Standard Model have properties that the neutrinos don’t: they have electric charges and also (sometimes) color charges, meaning they experience the electromagnetic and (sometimes) the strong nuclear interactions. The neutrinos, on the other hand, only experience the weak nuclear interaction, and so only appear in reactions that involve either a W-or-Z boson.
Although this occurs in many types of nuclear reactions that involve the weak force, that means that the primary way we produce neutrinos is either through fusion/fission reactions, or by creating another, heavier, unstable particle that will decay. These are the two primary ways to make neutrinos.

Regardless of whatever the particular underlying reaction is, however, they all have something in common:
- a subatomic particle interacts with another,
- involving a (real or virtual) W-or-Z boson,
- producing a neutrino (and other particles, too),
- which then goes off in a random direction.
You might read that last part and think, “A-HA! So it is all random!”
And that’s true, but there’s a caveat: it’s random with respect to what’s known in physics as the center-of-momentum (or, less accurately, the center-of-mass) rest frame. In nuclear fission reactors, where heavy nuclei are split apart and radioactive particles decay, the kinetic energies of the reactant particles are low compared to their masses, and so the frame-of-reference of the nuclear reactor itself is very close to the center-of-momentum frame itself. This is the reason why, when you look at the water (necessary for radiation shielding) that surrounds each nuclear reactor, you see that characteristic blue glow in all directions: because particles are emitted equally, in all directions, including massive charged particles that interact with the water itself.
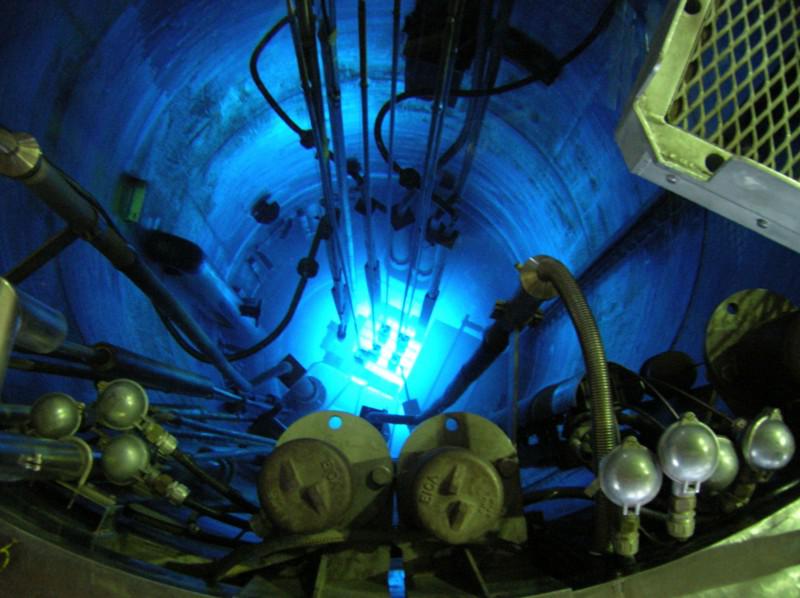
The very first neutrinos that were ever detected by humans were technically antineutrinos, detected by placing a specially made detector right next to the nuclear reactor itself. The proximity was of paramount importance, because the number of neutrinos you’ll have a chance to detect drops as the inverse of the distance squared: place your detector twice as far away and you’ll only capture one-quarter of the neutrinos you’d detect otherwise; place it ten times as far away and you’ll capture just one-hundredth of the neutrinos you’d capture from your closer location. The distribution of neutrinos spreads out just like light does: in a sphere, moving outward away from the source.
The difficulty inherent to detecting neutrinos isn’t restricted to nuclear reactors, either. In particle colliders, like the Large Hadron Collider at CERN, particles are smashed together at nearly the speed of light, with kinetic energies that are thousands of times the rest mass of the original particles. However, because the particles to be collided are circulated in opposite directions with equal (and opposite) momenta, these collisions also produce a random, evenly distributed set of daughter particles. When unstable particles like the Z-boson, W-boson, the charged pion, or heavy leptons like the muon or tau decay, they produce neutrinos as well; since they’re moving in random directions, so do the neutrinos.

In fact, of all the particles that arise from a collision in a large, powerful accelerator like the LHC, the neutrinos and antineutrinos are the only ones that aren’t captured by the detectors. (A small fraction of neutrinos actually are detected, but in most cases, an interaction between a neutrino and a particle in the detector never occurs.) Instead, when we reconstruct what occurred in the collision, we’re simply left with missing energy and momentum, and we can only infer the neutrinos and antineutrino properties by measuring what’s “missing” in order for energy and momentum to be conserved.
This might make you think that an experimental setup like DUNE, where neutrino beams are sent from a particle accelerator to a target that’s over 1000 kilometers (1300 km, in the case of DUNE) away, would be an absolute disaster. After all, if you’re creating neutrinos and antineutrinos, wouldn’t you want to put your neutrino detector as close as possible to the generating source? If the neutrinos are randomly distributed, and then their distribution spreads out spherically, wouldn’t it make more sense to put your detector mere meters away from the collision point, rather than over a million meters away?
Yet, that’s not what DUNE or (most) other neutrino experiments do, and there’s a remarkable set of reasons why.
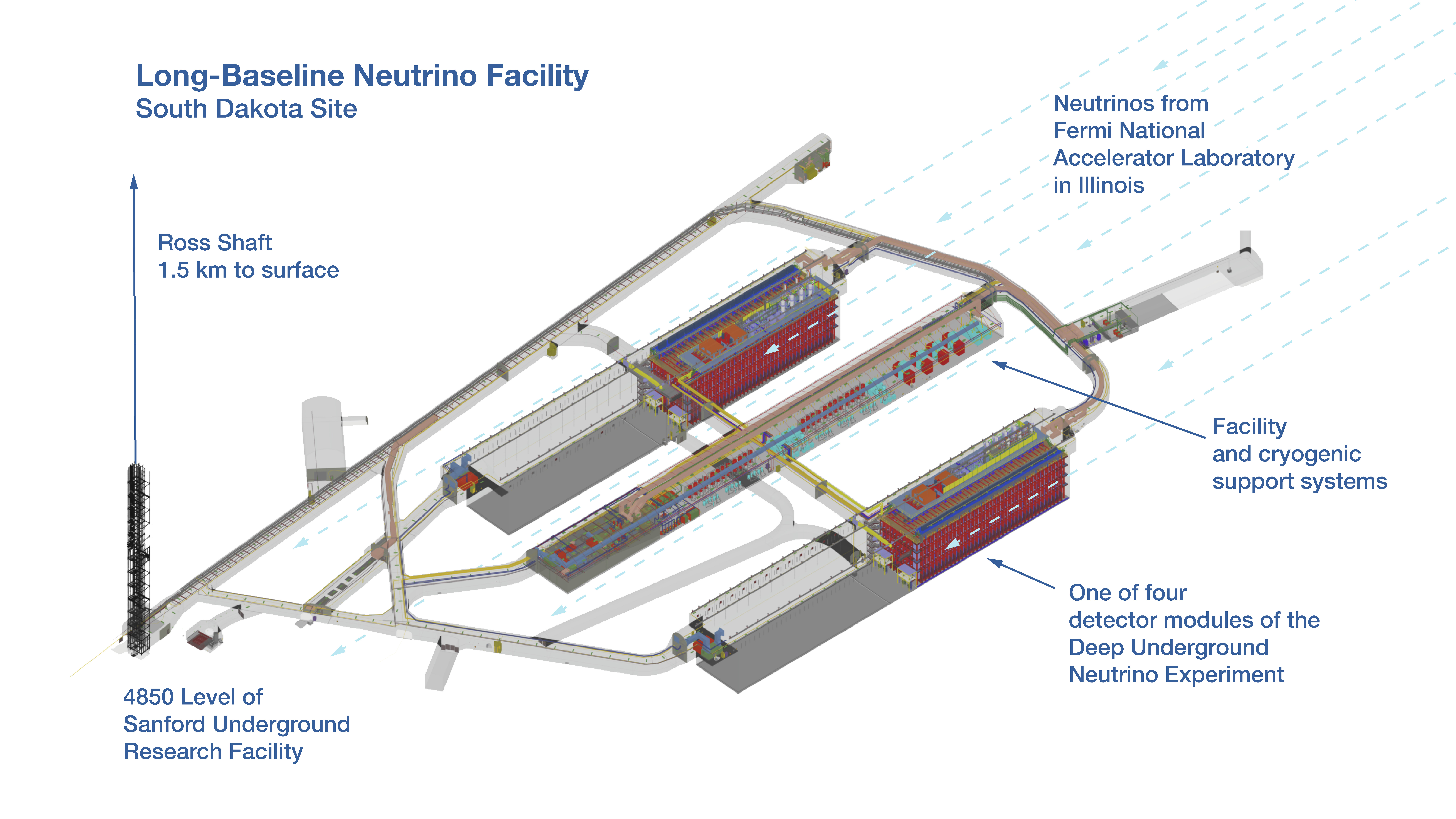
The first reason you don’t want to put a detector very close to the point at which you first create your neutrinos is that the neutrino signal, which is incredibly weak, would be absolutely swamped by all the other types of particles that would interact with your detector. In fact, in order to see neutrinos at all, you need to shield your detector from many of the other confounding signals that could appear in it. This typically involves building a large, shielded detector deep underground, where not only can accelerator-produced particles not get through, but even cosmic rays originating from the Sun, the atmosphere, and radioactive decays from the surrounding Earth are largely blocked. Only in these most-pristine of environments can the neutrino signal be precisely detected above the background noise.
The second reason you don’t want to put a detector very close to the neutrino creation point is because some of the most interesting properties of all, such as neutrino oscillations from one flavor into another, only occur when neutrinos travel through large amounts of space, and in particular, through matter. If you want to probe the properties of neutrinos and how they oscillate, or switch species mid-flight, you have to build your detector sufficiently far from the neutrino creation point so that they have ample opportunity to transition from one type to another.

But the third reason you want to build the detector far away from the neutrino creation point is because everything I told you earlier — about neutrinos being created in random directions, spreading out in a spherical distribution — only applies to the center-of-momentum frame. If, instead of colliding particles with a lot of kinetic energy into other particles moving with equal amounts of kinetic energy but moving in opposite directions, we collided particles with high kinetic energies into particles at rest, we’d get a narrowly collimated cone of particles: effectively, a beam!
The reason this occurs is because the center-of-momentum frame is now, relative to the rest-frame of the Earth (and the detector, and the collision point between the two colliding particles), heavily boosted. Yes, in that center-of-momentum frame, particles get created moving, with equal probability, in all directions. But then, to return back to the frame of reference of the laboratory, we have to boost that once again in the original direction of motion. What we get, then, is a stream of particles that moves very close to the speed of light in the direction that the pre-collisional particles were moving, with a little bit of “extra” motion superimposed atop it. The result is a solid cone of particles, ideally pointed in the direction of your eventual detector.
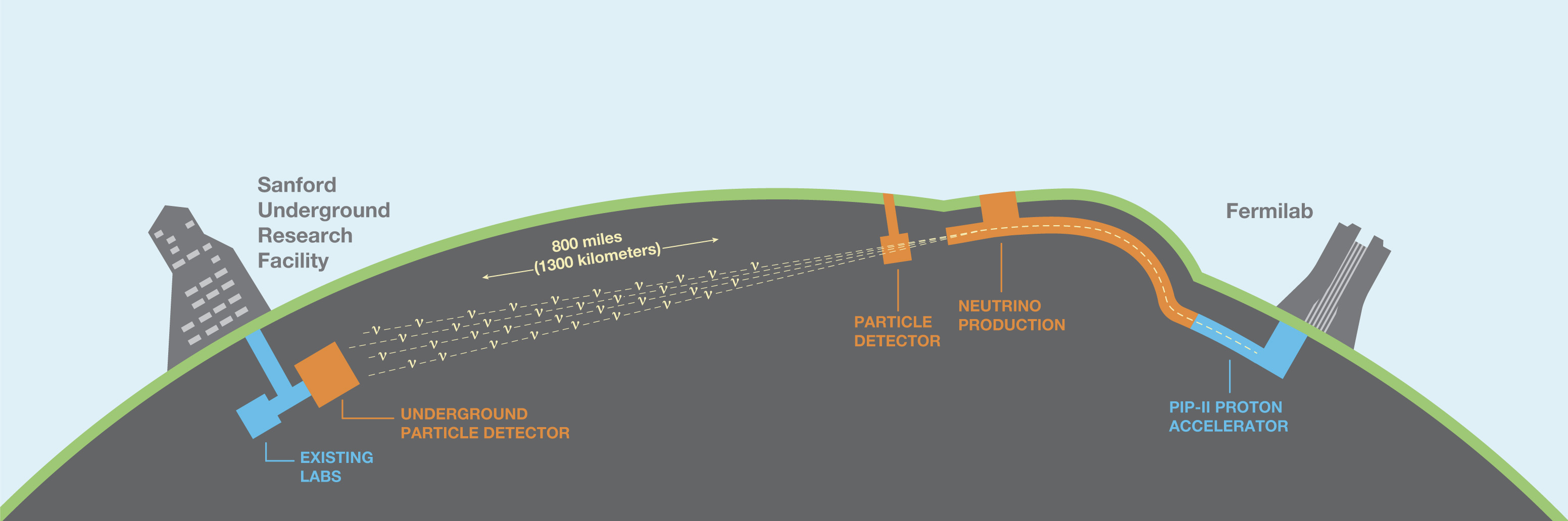
As far as transforming this beam of what particle physicists generically call “daughter particles” into a pure neutrino beam, physics takes care of that aspect all on its own, automatically. By accelerating protons to high energies — and they don’t need to be absurdly high; kinetic energies that are just a few times the rest mass energy of a proton will do just fine — the next step is to smash them into what we call a “fixed target,” which is a sacrificial piece of material that you don’t mind destroying by smashing high-energy protons into it. A block of acrylic or graphite, for example, will do just fine.
When protons strike atomic nuclei, they produce all sorts of particles, but the most common type of particle produced is a pion. Pions, for what it’s worth, are the lightest of all the unstable particles composed of quarks and/or antiquarks. Pions come in three varieties:
- The positively charged π+, which typically decays into an anti-muon and a muon neutrino after a few tens of nanoseconds,
- The neutral π0, which decays into two photons after only a fraction of a femtosecond (a million times smaller than a nanosecond), and
- The negatively charged π–, which typically decays into a muon and an anti-muon neutrino after a few tens of nanoseconds.
The muons (and antimuons), with a lifetime of 2.2 microseconds, then decay further: into an electron (or a positron), an anti-electron neutrino (or an electron neutrino), and a muon neutrino (or an anti-muon neutrino).
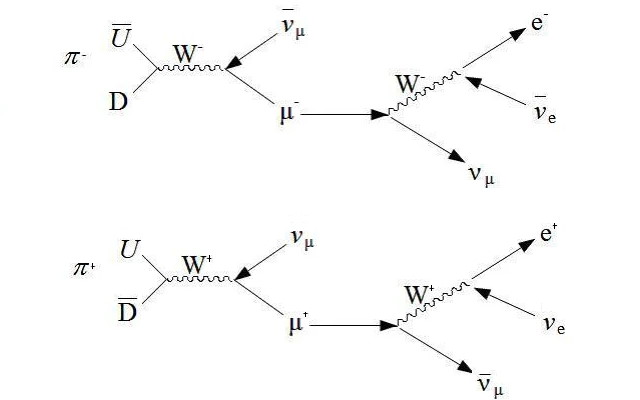
This is exactly what we want to happen, and ideally, we want all of these decays to happen before the makeshift “beam” we’ve created strikes the Earth. The Earth, you see, is the ultimate fixed target, as it easily collides with every particle that isn’t some type of neutrino or antineutrino. Meanwhile, the neutrinos and antineutrinos continue through relatively undisturbed; transforming a composite beam of particles and antiparticles into a neutrino/antineutrino beam alone simply requires firing your beam through the Earth. By collimating the charged particles with electromagnets before they decay, you can make the neutrino beam even narrower.
You can then put down a detector at the destination, where you detect a collimated beam of neutrinos and antineutrinos, but you can also put down a detector at any intermediate location along the way. With multiple different detection points, you can actually probe how neutrinos oscillate as they pass through the Earth. One of the science goals of DUNE is to search for any potential new neutrino physics, and this method makes us sensitive to the specific properties of the three known species of neutrino, plus any additional, exotic, sterile neutrinos that couple to the known ones. It’s a tremendously powerful way to probe the Universe, and DUNE, which will arguably be the most pristine detector of all-time, will answer many of the burning questions physicists presently have about these elusive particles.
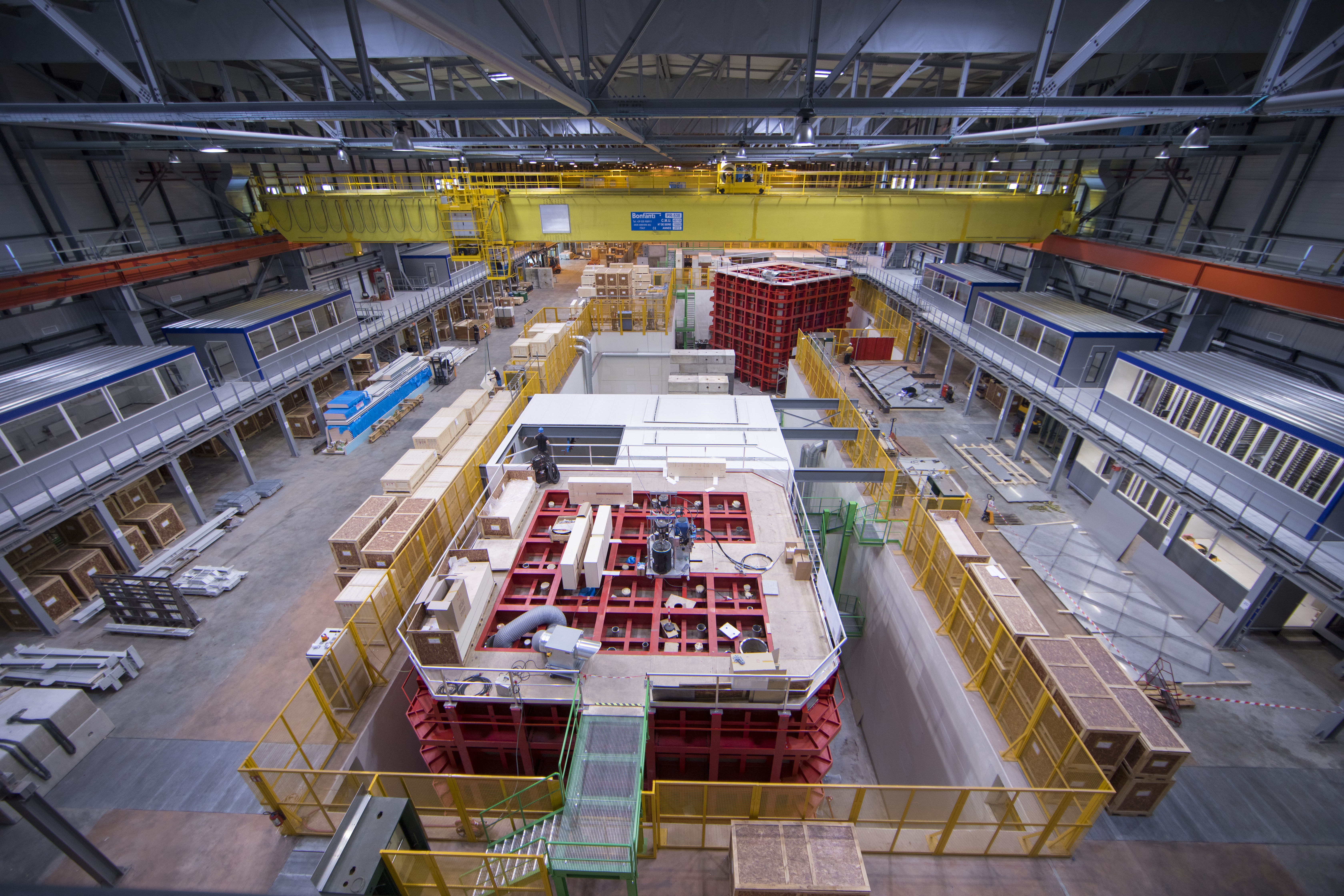
The added benefits that come from an enormous, pristine detector that’s underground is that you don’t simply get the signal arriving from the neutrino beam itself, but you also get:
- neutrinos that result from cosmic rays that strike our atmosphere,
- neutrinos that come from the Sun,
- neutrinos that may be arriving from supernovae, active galaxies, or black holes from far away,
- and the rare emissions of particles that result from natural radioactivity arising from the materials in and near the detector.
When you build a pristine detector to very high precision, you become sensitive to all sorts of interesting physical effects. The old saying among astronomers — that one astronomer’s noise is another astronomer’s data — applies equally well to neutrino physicists.
The ultimate goal of all of this is to probe the nature of fundamental particles, which involves measuring them in the greatest numbers possible to the highest precisions possible, while simultaneously acquiring the cleanest signal with the lowest levels of noise possible. When it comes to neutrino physics, we have the tremendous benefit of being able to finesse a beamline that can exceed even 1000 kilometers without having to clear a path for these ghostly particles. Just set up your experiment correctly and invest in your detectors, and the stopping power of planet Earth will guarantee you a cleaner signal than you’ll ever obtain anywhere else.
Send in your Ask Ethan questions to startswithabang at gmail dot com!