Ask Ethan: How are the heaviest elements of all made?
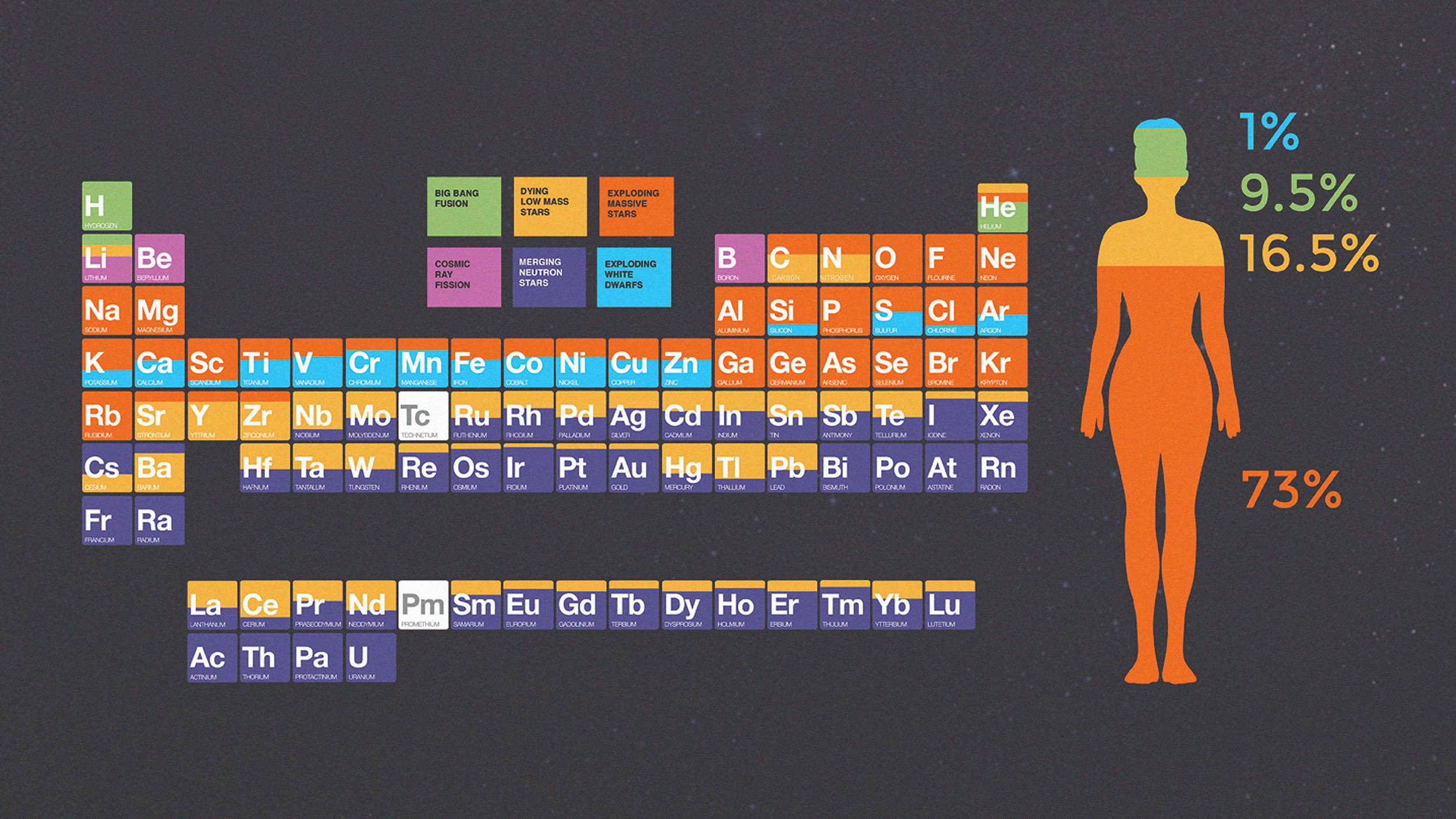
- Across our Universe, atoms and atomic nuclei are found everywhere, with hydrogen, helium, oxygen, and carbon making up the most abundant elements of all, in that order.
- While hydrogen and helium were forged in the Big Bang, practically all of the other elements are made in violent processes: in stars, supernovae, kilonovae, and more.
- But it’s the heaviest elements of all, the ones highest up on the periodic table, that have the most surprising origins. Here are the two ways those heaviest elements are made, and no, it’s not in supernovae.
Across the Universe, in all directions, there’s practically nowhere we can look that isn’t filled with some sort of matter: stars, galaxies, dust, gas clouds, and plasma chief among them, with small bodies scattered throughout each individual stellar system. When we examine this matter in detail, we find that it isn’t just made of the most common elements — hydrogen, helium, oxygen, carbon, and more — but that the full suite of elements found on the periodic table are found in various amounts all across the Universe. Here in our Solar System, we’ve been able to identify the abundance of these elements most precisely, but we also find them elsewhere: in and around newborn stars, in the interstellar medium, in supernova and kilonova remnants, in planetary nebulae, and much, much more.
But how, where, and when are these elements made? And, in particular, while the lighter elements are relatively easy to make, there are only two sources of production for the heaviest of all; why is that? That’s what Vince Tseng wants to know, asking:
“My question is on how the heaviest elements are created. I know that neutron star mergers are responsible for the synthesis of most heavier elements, but what are the physics behind this? And while we’re at it, why the [anomalies] for the heavy elements mercury, thallium, and lead? Why are substantial portions of those elements created by dying low mass stars, and what are the physics behind that?”
It’s a very deep question, and one we didn’t know the answer to until very recently. Here’s the physics and astrophysics behind the heaviest elements of all.

To be clear, there’s only one basic way to successfully transmute light elements into heavy elements: through the processes of nuclear fusion and of nuclear capture. You can either take two lighter atomic nuclei and compel them to react in an event that will lead to the production of a heavier atomic nucleus, or you can add a single nucleon (usually a neutron, but sometimes a proton) one-at-a-time to a pre-existing atomic nucleus. Either way, your original nucleus will now be heavier than the original nucleus, where it will then either:
- remain stable or quasi-stable,
- decay via the emission of an alpha particle (bringing it down two elements on the periodic table and reducing its mass by four atomic mass units),
- decay via the emission of a beta particle (keeping its atomic mass the same but bumping it up one element higher on the periodic table),
- or undergo a more exotic decay, which either keeps its atomic number the same or lowers it.
So, what are the various types of reactions that occur in the Universe that are capable of making these changes? Let’s go through each of them, and see where they do and don’t apply as far as creating the heaviest elements of all goes.

Fusion during the hot Big Bang.
It’s hard to believe, looking at our rich Universe today, but in the earliest stages of the hot Big Bang, there were no atoms, no heavy elements, and in fact nothing more complex — as far as an atomic nucleus goes — than bare protons and neutrons. With the very high temperatures and ultra-high densities present in the early Universe, you might think this would’ve been the ideal conditions for forging heavy elements: all the way up the periodic table. It’s hard to fault you if you think this way; that’s what George Gamow, arguably the most famous name associated with the Big Bang, once thought, too!
The problem, however, is that there are so many photons, or quanta of light, present in the early Universe that they prevent the first composite atomic nucleus, deuterium, from stably forming for long periods of time. Each time a deuteron (a proton and neutron combined) forms, a high-enough energy photon comes along and blasts it apart, delaying all further steps that would build up heavy elements. By the time the Universe cools enough that deuterium can stably form, passing what’s known as the deuterium bottleneck, temperatures are still high, at billions of degrees, but densities are low: just one-billionth of what they are in the Sun’s core. As a result, only the lightest elements are produced in any sort of abundance: hydrogen and helium, along with their various isotopes. Lithium is created at less than the one-part-per-billion level, and beryllium, boron, carbon, nitrogen, and oxygen are all progressively even less abundant: at the parts-per-trillion or parts-per-quadrillion level.
For heavy element production, the Big Bang won’t do at all.

Fusion inside normal, main-sequence stars.
So, you want to make heavy elements? The most abundant place in our modern Universe where heavy elements are forged is exactly where you’d expect: inside the cores of normal stars. As long as the core of your hydrogen-rich ball of gas and plasma can reach temperatures exceeding 4,000,000 K, you’re going to trigger nuclear fusion reactions: primarily through the proton-proton chain at lower temperatures, while higher temperatures (found in the cores of more massive stars) will produce heavier elements primarily through the C-N-O cycle. For as long as stars are on the main sequence — i.e., that they haven’t yet evolved into giant stars — these are the processes that dominate.
Unfortunately for you heavy element fans, the primary reactions that occur inside these main-sequence stars only wind up with their net reactions forming helium out of hydrogen; heavier elements aren’t produced in these environments. Even in the C-N-O cycle, involving carbon, nitrogen, and oxygen, there is no net production of other elements: just hydrogen into helium, plus electrons, positrons, and gamma-rays: all of which do nothing to create still-heavier elements. However, main-sequence stars come in three varieties:
- high-mass stars, with more than 8-10 solar masses,
- normal stars, with between 0.4 and 8 solar masses,
- and low-mass stars, with 0.4 or less solar masses.
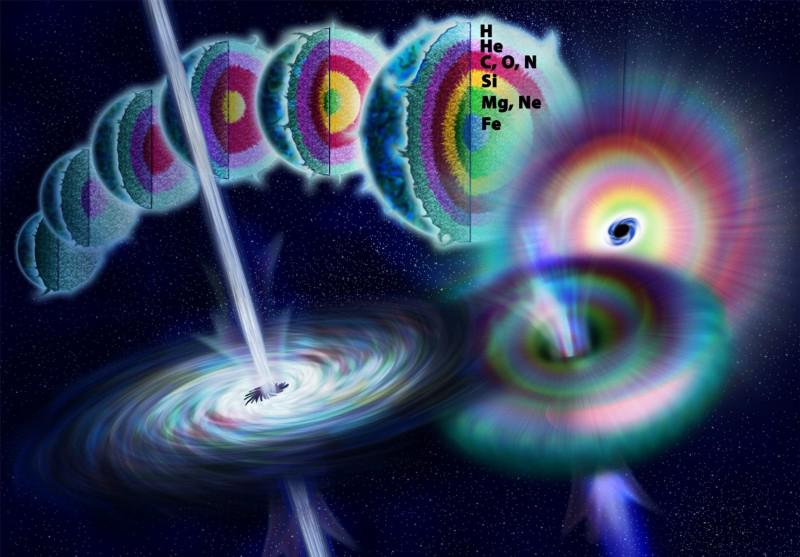
The low-mass stars will never leave the main sequence, so they’ll never make anything heavier than helium through nuclear reactions. But the other two types are worth a closer look as they evolve.
Fusion inside giant, massive, evolved stars.
This one is more fun: when a massive star burns through the hydrogen in its core, what happens next? That core, without further hydrogen fuel within it, begins to contract and heat up. As it heats up, new sets of reactions become possible at higher temperatures: the fusion of helium nuclei into carbon. While hydrogen will fuse into helium in a shell around the inner core, the core itself moves on to heavier elements. Once the core helium is exhausted, this contraction process reappears, as:
- carbon fuses into neon, oxygen, sodium, and magnesium,
- then neon fuses into oxygen and magnesium,
- then oxygen fuses into silicon, sulphur, phosphorous, and magnesium,
- then silicon fuses into (primarily) sulphur, argon, calcium, titanium, chromium, and eventually into iron, nickel, and cobalt,
and that’s it.
However, as a by-product of some of the reactions that occur, free neutrons are produced. These neutrons can be captured by atomic nuclei, allowing a slow build-up of heavier elements in the cores of these stars: where the neutrons are produced. The slow nature of the reaction (slow neutron-capture process) is why this is called the s-process, and can easily create elements up to the iron-cobalt-nickel plateau: the most energetically stable elements of all. However, it’s difficult to go significantly above these elements, because of the large numbers of mid-mass elements and the dearth of neutrons produced relative to the overall star. To climb higher in any great abundance, more energy is needed.

Core-collapse supernova events.
Fortunately, when silicon-burning completes, that’s exactly what occurs most of the time: the core, now inert (as iron atoms won’t fuse together), contracts and implodes, triggering a runaway fusion reaction. A core of either a neutron star or a black hole is produced, while the outer layers are blasted away in a stellar cataclysm: a core-collapse (or type II) supernova event. Large numbers of neutrons are rapidly produced over a span of several seconds, enabling many neutrons to be captured at once: an example of a rapid neutron-capture process, or r-process, as opposed to the slower and more enduring s-process.
You might think, “Great, large numbers of neutrons all at once, that ought to give us elements as heavy as we desire!” And it’s a nice thought, but it’s not correct. We can verify this by looking at the elements produced by core-collapse supernovae through a thorough examination of their remnants. What we find is that these supernova events:
- produce large amounts of all elements from carbon (element 6) up through rubidium (element 37),
- then small amounts of elements strontium, yttrium, and zirconium (elements 38, 39, and 40),
- but practically no elements beyond that,
although there is some suggestive evidence that a little bit of elements such as tellurium, iodine, and xenon can be produced this way. Supernovae may create most of the heavy elements in the Universe, but for the heaviest elements of all, we just don’t see the production we might hope for in core-collapse events.

Credit: Sakurambo/SkateBiker at English Wikipedia
Fusion inside normal, highly evolved stars.
What about normal, Sun-like stars, then, once they evolved off of the main sequence and begin fusing helium in their cores? This is kind of a “sweet spot” for making many of the heaviest elements of all. Helium fusion occurs in these stars over long periods of times: from hundreds of millions to even billions of years. When it does occur, we don’t just get three helium atoms fusing to make carbon; we also get a little bit of carbon-13 fusing with helium, which produces oxygen and a free neutron, as well as neon-22 fusing with helium, producing magnesium and a free neutron.
Where do these free neutrons go? That’s the s-process appearing once again, where this time, the star is often well-mixed: with all sorts of elements, not just hydrogen, helium, carbon, neon, and oxygen present throughout it. Instead, it now becomes possible to build elements all the way up the periodic table: from strontium (element 38) through lead (element 82), simply by:
- adding neutrons one at a time,
- making your atomic nucleus heavier (but keeping it the same element),
- until your nucleus is so heavy that it becomes unstable against beta decay,
- which transmutes a neutron in your nucleus into a proton,
bumping you up to one element higher on the periodic table. This only produces heavy elements slowly, but it does so steadily. If not for the fundamental instability of polonium (element 84), we could theoretically go higher, but instead, lead-and-bismuth marks the end of the line for this method of production.
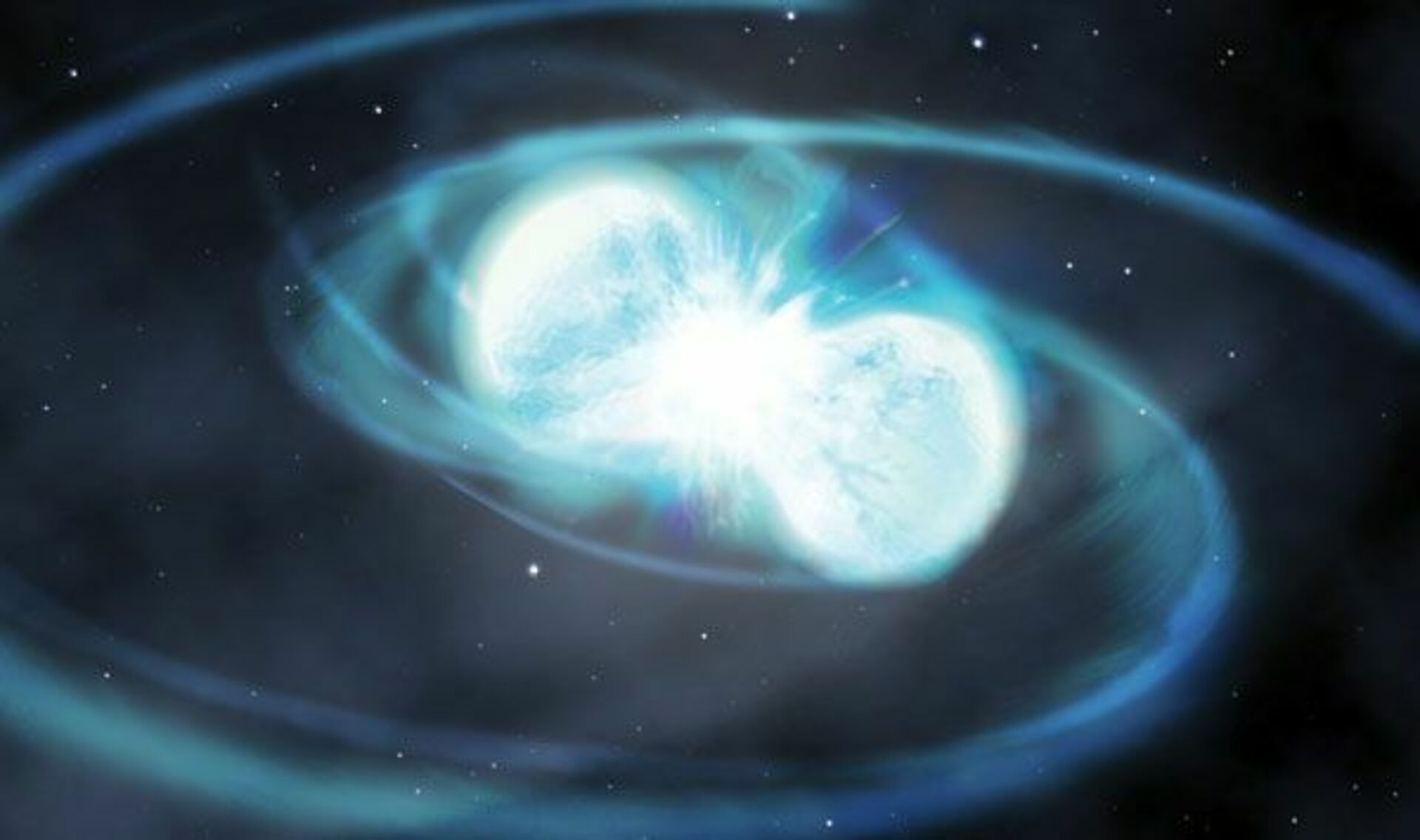
Credit: D. A. Howell, Nature, 2010
Type Ia supernova (exploding white dwarf) events.
“Ah ha,” you think, “when two white dwarfs collide, a runaway nuclear fusion reaction occurs, triggering a type Ia supernova event.” This is true! But, just as with other supernova events, we can look at the remnants arising from those supernovae, and see what sorts of elements are found throughout them, distributed practically everywhere.
Fusion reactions do copiously occur in these supernova events, but they don’t produce heavy elements at all; they’re the reactions that are most responsible for the production of elements such as:
- titanium,
- vanadium,
- chromium,
- manganese,
- iron,
- cobalt,
- nickel,
- copper,
- and zinc.
However, this process fails to make a substantial abundance of elements heavier than this, as there is neither a rapid nor slow production of copious amounts of neutrons; there’s just the fusion reactions that occur in one major burst. Although it may have been theoretically possible to imagine a scenario where either type Ia supernovae or type II supernovae produced these heaviest of elements in significant abundance, this simply isn’t what we find from examining the actual events that occur within our Universe.
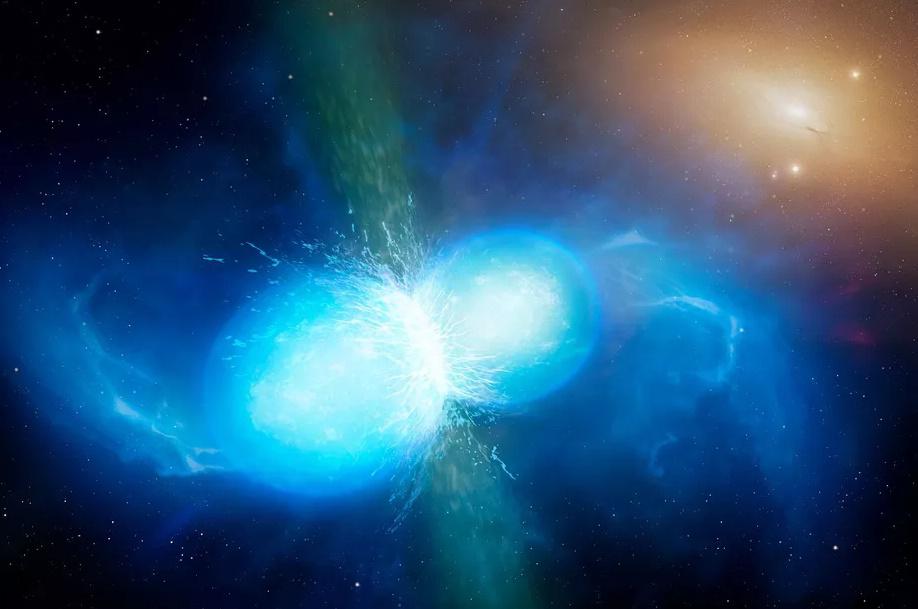
Kilonova (colliding neutron star) events.
But kilonovae, which occur when two neutron stars collide, can make these elements. We had observed bright, energetic bursts in the sky — gamma-ray bursts — that could be localized, and when they were, they displayed signatures of a great variety of the heaviest elements of all: all the way up the periodic table, including to unstable, highly radioactive elements such as uranium, plutonium, and curium. How were these elements made?
From the merger of two neutron stars, as we were first able to confirm with a 2017 event that showed:
- gravitational waves from an inspiral and merger,
- a gamma-ray burst from the merger-driven explosion,
- an afterglow across all wavelengths of light,
- and the surefire signature of heavy element production thereafter,
all correlated with the same astrophysical event. Subsequent observations of additional kilonova events, including with spectroscopic follow-up, have revealed this type of heavy element production from all across the Universe. Apparently, having a heavy element-rich crust atop an interior of neutrons means that, when two such objects collide, large quantities of heavy elements are easily produced; with only a few exceptions where the s-process in dying Sun-like stars dominates, most of the heaviest elements of all are produced in neutron star-neutron star mergers.

And that’s it! Of all the ways to make heavier elements out of lighter ones, these are the only two cosmic ways, as far as we understand the Universe at present, to make elements heavier than zirconium (which is only element #40) on the periodic table: through kilonovae and through the slow neutron capture process (s-process) in low-mass, evolved, giant stars. Additionally, the s-process can only build you up to element #83, bismuth, on the periodic table, since adding a neutron to bismuth, creating polonium, leads to a relatively rapid alpha-decay, bringing us back down to lead: element #82. All heavier elements, therefore, should only be created in kilonova events.
Back in 2017, we observed our first neutron star-neutron star collision directly in gravitational waves, which came along with a gamma-ray signature and an identifiable remnant as well. However, in all the time since, we have yet to make a similar detection: of a neutron star-neutron star merger in gravitational waves that also resulted in a kilonova. Are there truly enough kilonovae to account for the observed abundance of these heavy elements? We still aren’t certain, except to say that if there is another source for these ultra-heavy elements, we have no idea what it could be. The heaviest elements should come from colliding neutron stars, with a slight assist from the slow neutron capture process in giant, evolved, Sun-like stars, and those are the only known plausible sources at present.
Send in your Ask Ethan questions to startswithabang at gmail dot com!