How All Of Physics Exists Inside A Single Atom
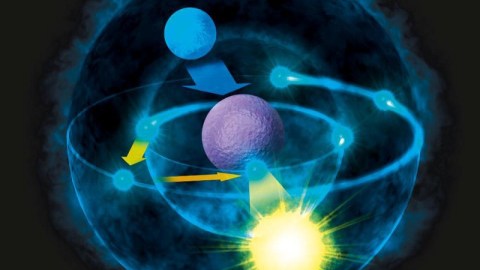
Using atoms to probe the Universe reveals the complete Standard Model.
If you wanted to uncover the secrets of the Universe for yourself, all you’d have to do is interrogate the Universe until it revealed the answers in a way you could comprehend them. When any two quanta of energy interact — irrespective of whether they’re particles or antiparticles, massive or massless, fermions or bosons, etc. — the result of that interaction has the potential to inform you about the underlying laws and rules that the system has to obey. If we knew all the possible outcomes of any interaction, including what their relative probabilities were, then and only then would we claim to have some understanding of what was going on.
Quite surprisingly, everything that we know about the Universe can, in some way, be traced back to the most humble of all the entities we know of: an atom. An atom remains the smallest unit of matter we know of that still retains the unique characteristics of the macroscopic world, like physical and chemical properties. And yet, it’s a fundamentally quantum entity, with its own energy levels, properties, and conservation laws. Moreover, even the humble atom couples to all four of the known fundamental forces. In a very real way, all of physics is on display, even inside a single atom. Here’s what they can tell us about the Universe.
Here on Earth, there are approximately ~90 elements that occur naturally: left over from the cosmic processes that created them. An element is fundamentally an atom, with an atomic nucleus made of protons and (possibly) neutrons and orbited by a number of electrons that’s equal to the number of protons. Each element has its own unique set of properties, including:
- hardness,
- color,
- melting and boiling points,
- density (how much mass occupied a given volume),
- conductivity (how easily its electrons are transported when a voltage is applied),
- electronegativity (how strongly its atomic nucleus holds onto electrons when bound to other atoms),
- ionization energy (how much energy is required to kick an electron off),
and many others. What’s remarkable about atoms is that there’s only one property that defines what type of atom you have (and hence, what these properties are): the number of protons in the nucleus.
Given the diversity of atoms out there and the quantum rules that govern the electrons — identical particles — that orbit the nucleus, it’s not hyperbole at all to make the claim that everything under the Sun is truly made, in some form or other, of atoms.
Every atom, with its unique number of protons in its nucleus, will form a unique set of bonds with other atoms, enabling a practically unlimited set of possibilities for the types of molecules, ions, salts, and larger structures that it can form. Primarily through the electromagnetic interaction, the subatomic particles that compose atoms will exert forces on one another, leading — given enough time — to the macroscopic structures we observe not only on Earth, but everywhere throughout the Universe.
At their very core, however, atoms all have the property of being massive in common with one another. The more protons and neutrons in the atomic nucleus, the more massive your atom is. Even though these are quantum entities, with an individual atom spanning no more than a single ångström in diameter, there’s no limit to the range of the gravitational force. Any object with energy — including the rest energy that gives particles their masses — will curve the fabric of spacetime according to Einstein’s theory of General Relativity. No matter how small the mass, or how small the distance scales are that we work with, the curvature of space induced by any number of atoms, whether ~10⁵⁷ (like in a star), ~10²⁸ (like in a human being), or just one (like in a helium atom), will occur exactly as the rules of General Relativity predict.
Atoms are also made up of electrically charged particles. Protons have a positive electric charge inherent to them; neutrons are electrically neutral overall; electrons have an equal-and-opposite charge to the proton. All of the protons and neutrons are bound together in an atomic nucleus just a femtometer (~10^-15 m) in diameter, while the electrons orbit in a cloud that’s some 100,000 times larger in size. Each electron occupies its own unique energy level, and electrons can only transition between those discrete energies; no other transitions are allowed.
This is remarkable in two different ways. On the first hand, when an atom comes into the vicinity of another atom (or group of atoms), they can interact. At a quantum level, their wavefunctions can overlap, allowing atoms to bind together into molecules, ions, and salts, with these bound structures having their own unique shapes and configurations for their electron clouds. Correspondingly, they also have their own unique energy levels, which absorb and emit photons (particles of light) only of a particular set of wavelengths.
These electron transitions within an atom or group of atoms are unique: particular to the atom or the configuration of a group of multiple atoms. When you detect a set of spectral lines from an atom or molecule — whether they’re emission or absorption lines doesn’t matter — they immediately reveal what type of atom or molecule you’re looking at. The internal transitions of the electrons gives a unique set of energy levels, and the transitions of those electrons reveal unambiguously what type and configuration of atom(s) you have.
From anywhere in the Universe, atoms and molecules obey these same rules: the laws of classical and quantum electrodynamics, which govern every charged particle in the Universe. Even inside the atomic nucleus itself, which is internally composed of (charged) quarks and (uncharged) gluons, the electromagnetic forces between these charged particles is tremendously important. This internal structure explains why the magnetic moment of a proton is almost three times the magnitude of the electron’s magnetic moment (but of opposite sign), while the neutron has a magnetic moment that’s almost twice as large as the electron’s, but the same sign.
While the electric force has a very long range — the same, infinite range as gravitation, in fact — the fact that atomic matter is electrically neutral as a whole plays a tremendously important role in understanding how the Universe we experience behaves. The electromagnetic force is fantastically large, as two protons will repel each other with a force that’s ~10³⁶ times larger than their gravitational attraction!
But because there are so many atoms making up the macroscopic objects we’re used to, and atoms themselves are electrically neutral overall, we only notice when either:
- something has a net charge, like a charged-up electroscope,
- when charges flow from one location to another, like during a lightning strike,
- or when charges get separated, creating an electric potential, such as in a battery.
One of the simplest and most fun examples of this comes from rubbing a blown-up balloon on your shirt, and then attempting to stick the balloon either to your hair or to the wall. This works only because the transfer or redistribution of a small number of electrons can cause the effects of a net electric charge to completely overcome the force of gravity; these van der Waals forces are intermolecular forces, and even objects that remain neutral overall can exert electromagnetic forces that — over short distances — can themselves overcome the power of gravity.
At both a classical and quantum level, an atom encodes a tremendous amount of information about the electromagnetic interactions in the Universe, while “classical” (non-quantum) General Relativity is completely sufficient to explain every atomic and subatomic interaction we’ve ever observed and measured. If we venture even further inside the atom, however, to the interior of the protons and neutrons inside the atomic nucleus, we can reveal the nature and properties of the remaining fundamental forces: the strong and weak nuclear forces.
As you venture down to ~femtometer scales, you’ll first start to notice the effects of the strong nuclear force. It first shows up between the different nucleons: the protons and neutrons that make up each nucleus. Overall, there’s an electric force that either repels (since two protons both have like electric charges) or is zero (since neutrons have no net charge) between the different nucleons. But at very short distances, there’s an even stronger force than the electromagnetic force: the strong nuclear force, which occurs between quarks through the exchange of gluons. Bound structures of quark-antiquark pairs — known as mesons — can be exchanged between different protons and neutrons, binding them together into a nucleus and, if the configuration is right, overcoming the repulsive electromagnetic force.
Deep inside these atomic nuclei, however, there’s a different manifestation of the strong force: the individual quarks inside are continuously exchanging gluons. In addition to the gravitational (mass) charges and the electromagnetic (electrical) charges that matter possesses, there’s also a type of charge specific to the quarks and gluons: a color charge. Instead of being always positive and attractive (like gravity) or negative and positive where like charges repel and opposites attract (like electromagnetism), there are three independent colors — red, green, and blue — and three anti-colors. The only allowable combination is “colorless,” where all three colors (or anticolors) combined, or a net colorless color-anticolor combination are permitted.
The exchange of gluons, particularly when quarks get farther apart (and the force gets stronger), is what holds these individual protons and neutrons together. The higher the energy that you smash something into these subatomic particles, the more quarks (and antiquarks) and gluons you can effectively see: it’s like the inside of the proton is filled with a sea of particles, and the harder you smash into them, the “stickier” they behave. As we go to the deepest, most energetic depths we’ve ever probed, we see no limit to the density of these subatomic particles inside every atomic nucleus.
But not every atom is going to last forever in this stable configuration. Many atoms are unstable against radioactive decay, meaning that eventually they will spit a particle (or a set of particles) out, fundamentally changing the type of atom that they are. The most common type of radioactive decay is alpha decay, where an unstable atom spits out a helium nucleus with two protons and two neutrons, which relies on the strong force. But the second most common type is beta decay, where an atom spits out an electron and an anti-electron neutrino, and one of the neutrons in the nucleus transforms into a proton in the process.
This requires yet another novel force: the weak nuclear force. This force relies on a wholly new type of charge: weak charge, which itself is a combination of weak hypercharge and weak isospin. The weak charge has proven tremendously difficult to measure, since the weak force is millions of times smaller than either the strong force or the electromagnetic force until you get down to extraordinarily small distance scales, like 0.1% the diameter of a proton. With the right atom, one that’s unstable against beta decay, the weak interaction can be seen, meaning that all four of the fundamental forces can be probed simply by looking at an atom.
This also implies something remarkable: that if there’s any particle in the Universe, even one we have yet to discover, that interacts through any of these four fundamental forces, it will also interact with atoms. We’ve detected a great many particles, including all the different types of neutrinos and antineutrinos, through their interactions with the particles found within the humble atom. Even though it’s the very thing that makes us up, it’s also, in a fundamental way, our greatest window into the true nature of matter.
The farther inside the building blocks of matter we look, the better we understand the very nature of the Universe itself. From how these various quanta bind together to make the Universe we observe and measure to the underlying rules that every particle and antiparticle obeys, it’s only by interrogating the Universe that we have that we can learn about it. As long as the science and technology we’re capable of constructing is capable of investigating it further, it would be a pity to give up on the search simply because a new, paradigm-shattering discovery isn’t guaranteed. The only guarantee we can be certain of is that if we fail to look more deeply, we won’t find anything at all.
Starts With A Bang is written by Ethan Siegel, Ph.D., author of Beyond The Galaxy, and Treknology: The Science of Star Trek from Tricorders to Warp Drive.