The true reason why Einstein was history’s greatest physicist
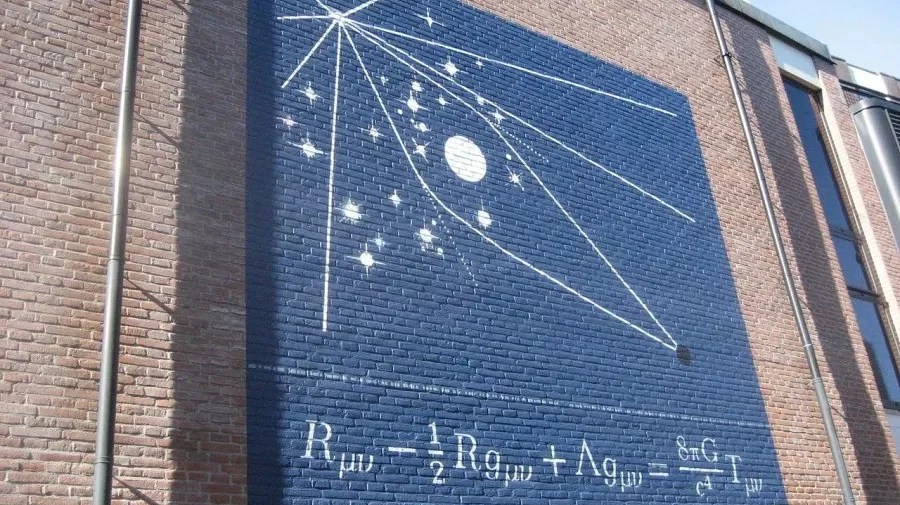
- Albert Einstein wasn’t just a scientific revolutionary, he was a celebrity and cultural icon throughout most of his life in the 20th century.
- Famed for the relativity of motion, the constancy of the speed of light, E = mc², and winning the Nobel Prize for the photoelectric effect, he also brought us mind-bending ideas like wormholes and the EPR paradox.
- But it’s his general theory of relativity, put forth in 1915, that sets him apart from everyone else in the minds of most professional physicists. Over 100 years later, it’s still flawless.
Although many people struggle to name even one living scientist, practically everyone around the world knows who Einstein was. Perhaps the most famous person of the 20th century, Einstein revolutionized the sciences of physics and astronomy, making important contributions that taught us:
- the speed of light is the same for everyone,
- that energy and mass are equivalent, and related by the equation E = mc²,
- that light and electrons are both quantized into discrete “packets” of energy,
- and that space and time are not absolute and objective,
among many other discoveries that are still relevant today. Einstein’s work continues to endure on a number of other fronts as well, including on paradoxes in quantum entanglement (the EPR paradox), on connecting two well-separated points in spacetime through wormholes (Einstein-Rosen bridges), and in describing the statistics of integer-spin particles (Bose-Einstein statistics).
That would have been enough for a remarkable career and a place among the all-time greats in physics. But the crown jewel of Einstein’s achievements was his revolutionary new theory of gravity — general relativity — which was somehow put forth all the way back in 1915 and has succeeded in every prediction it’s made in all the time since. No other physical theory has endured, without fail, for so long. It’s this success, above all others, that explains why physicists revere and esteem Albert Einstein so highly. Let’s explore why.

The problem of Newton’s gravity
Although Newton’s gravity was first put forth way back in the 1600s, it seemed to explain absolutely everything we observed — in the heavens and on Earth — for around the next 200 years. For a while, it looked as though something was odd with Uranus’s orbit, as it wasn’t quite obeying Kepler’s laws of planetary motion as expected, from it’s discovery in 1781:
- it initially appeared to orbit quicker than expected for the first ~20 years after its discovery,
- then appeared to slow down and orbit at the predicted speed for the next ~20 years,
- and then slowed down further, orbiting too slowly for the ~20 years thereafter.
While some suspected a flaw with Newton’s gravity, the culprit turned out to be an eighth, more distant planet gravitationally tugging on Uranus: Neptune. Its 1846 discovery quieted any critics that Newton had out there.
But a new problem wasn’t so easy to get rid of: the orbit of Mercury, whose perihelion was precessing at a slightly greater rate than Newton’s law of universal gravitation could account for. Additionally, Newton’s laws of motion couldn’t account for behaviors that arose near the speed of light, such as length contraction and time dilation. Newton’s laws, including the laws of motion and the law of gravitation, appeared to work very, very well under nearly all circumstances, but these exceptions were perhaps a hint of something new, exciting, and revolutionary that would take us beyond Newton’s conception of the Universe.

Einstein’s relative ideas
In the middle of the 19th century, a seemingly unrelated scientific revolution was occurring: the discovery of the electromagnetic forces, fields, and how they behaved. One of the more interesting things that came out of electromagnetism was the understanding that light itself is an electromagnetic wave: with oscillating, in-phase electric and magnetic fields that propagated at perpendicular directions to one another. Light had a propagation speed — the speed of light in a vacuum — and experiments conducted to measure the speed in mutually perpendicular directions, including with and against the motion of the Earth around the Sun, always reached the same conclusion: that the speed of light never appeared to change.
How could this be? How could light move at the same speed irrespective of whether:
- it was emitted along with Earth’s motion,
- it was emitted against Earth’s motion,
- it was emitted from a source that was stationary with respect to Earth,
- or it was emitted in a direction perpendicular to Earth’s motion?
Einstein tried to think of a circumstance where one could follow a light wave and see these electric and magnetic fields oscillating in perpendicular directions with one another, and concluded that it never occurred in nature. The speed at which light appeared to move, he realized, would be the same for all observers. It was neither space nor time that was absolute and agreed upon by everyone, but the speed of light that was the invariant quantity. From this insight, special relativity was born, and with it, Newton’s laws of motion would only apply to non-relativistic motion, not to speeds approaching the speed of light.

The happiest thought of his life
But Einstein wasn’t done, not by a long shot. In 1907, his former professor Hermann Minkowski wove three-dimensional space and one-dimensional time into a four-dimensional fabric known as spacetime, where both space and time were relative to each observer, but a certain quantity — today known as the spacetime (or Einstein) interval — is always left invariant and unchanging. While thinking about an object’s motion through spacetime, Einstein had a realization that he would later call his “happiest thought:” the equivalence principle.
He was considering the motion of a room through spacetime, and the two cases of:
- the room accelerating due to the influence of an outside force,
- or the room being stationary, at rest, on the surface of the Earth,
when he realized that a person in the center of each room who dropped a ball would see, feel, and experience exactly the same physical influences. The two situations were physically equivalent, and hence, gravitation was just another form of acceleration. It was this key physical insight that led Einstein to abandon the flat, uncurved spacetime of special relativity in favor of developing a curved spacetime: where matter-and-energy determined the curvature of space, and that curved space then would, in turn, determine how matter-and-energy moved through it. By the end of 1915, Einstein had succeeded, and put his new theory, general relativity, out into the world in its final, modern form.

Putting Einstein to the test
While theoretical developments happened quickly within general relativity, the critical, needed test would be observational. Sure, Einstein had already shown that:
- in weak gravitational fields, his theory would recover Newton’s laws,
- in the absence of gravitational fields, his theory would reduce to special relativity,
- at low speeds, his equations of motions would once again be Newtonian,
- and that his theory could account for observations, like the precession of Mercury’s perihelion, that Newton’s laws could not.
Those steps were all necessary in order for his new theory to remain viable, as any new theory must both reproduce all the successes of the old one and also explain something important that the old theory can’t.
However, general relativity would have to clear a third hurdle as well: making a new, yet-untested prediction that differed from the old (Newtonian) theory’s predictions, that could then go out and be tested. That prediction came in the form of the bending of starlight due to gravity: something that could be tested for the positions of stars located close to the Sun during a total solar eclipse. While the Great War made a 1916 test impossible and clouds ruined an attempt to measure 1918’s eclipse in the United States, the total solar eclipse of 1919 settled the matter: Einstein’s predictions were correct and in agreement with observations, while the Newtonian predictions were ruled out. At long last, we had a new theory of gravity to describe our Universe.

An expanding Universe
Einstein’s general relativity, although an incredibly powerful theory, isn’t the easiest theory to work with or extract predictions from. It’s incredibly mathematically intensive to work with, and that makes extracting quantitative predictions difficult, to say the least. Furthermore, it’s very important that we model whatever physical system we’re considering correctly, in that we have to write down the correct spacetime for the problem we’re considering. (Which makes solving problems that much more difficult.) It’s why even though there are, theoretically, an infinite number of solutions to Einstein’s equations, there is only one physical Universe to apply them to.
Which is why it was such a surprise to everyone, Einstein included, when Georges Lemaître sent Einstein a letter in 1927, showing him that if you combined the spacetime solution for a Universe that was uniformly filled with matter-and-energy (which Einstein had erroneously called “incompatible” with his field equations in 1922) with the data for distances to and redshifts of galaxies, you could conclude that the Universe was expanding. Einstein did not believe this was possible, and retorted, “Vos calculs sont corrects, mais votre physique est abominable,” which translates to, “Your calculations are correct, but your physics is abominable.”
But Lemaître was correct, as Howard Robertson showed the next year and then Hubble showed more definitively the year after. The Universe really is expanding, and Einstein’s theory provided the theoretical explanation as to how, why, and by how much.

Gravitational redshift
There are three main physical phenomena that explain why light can sometimes redshift, or get shifted to longer wavelengths, within the Universe.
- There’s a Doppler shift, just like there is for sound waves, that arises owing to the relative motion of the emitting source and the observer who detects the signals emitted from that source.
- There’s the cosmological shift, determined by the expansion of space between the source and the observer, which lengthens the light’s wavelength as it travels throughout the expanding Universe.
- And there’s also gravitational redshift (or blueshift), where climbing out of a gravitational field (from a more curved to a less curved region) or falling into a gravitational field (to a region of greater curvature) causes the wavelength of light to either lengthen (for redshift) or shorten (for blueshift) from purely gravitational effects.
This is observed, astrophysically, for light from the cosmic microwave background from both the Sachs-Wolfe effect and the integrated Sachs-Wolfe effect, but was first demonstrated in a laboratory experiment that involved sending light from lower down in Earth’s gravitational field to higher up: 1960’s Pound-Rebka experiment. Even after Einstein’s death, previously untested predictions of his theory continued to be experimentally verified, including to precisions unimaginable to Einstein during his life.

Black holes
Perhaps the most remarkable prediction of general relativity is the existence of black holes: of regions of space where so much energy is compacted into such a small volume of space that an event horizon forms. From within that region bounded by the event horizon, no object, not even light itself, can escape. Predicted to arise, astrophysically, from either the collapse of matter or the implosion of a stellar corpse, Einstein’s theory makes a series of fascinating predictions for black holes and the spacetime surrounding them, both in the rotating and non-rotating cases.
Black holes should:
- cause tidal disruption events, when stars or other forms of matter pass too close to one,
- cause luminous objects, such as stars, white dwarfs, or neutron stars, to appear to orbit a non-luminous but massive point in space,
- siphon mass off of less dense companions, creating accretion disks and generating X-ray emissions,
- and emit radio-wave light from their surrounding environs, which should appear “dark” as it’s silhouetted by the black hole’s event horizon “shadow.”
All of these predictions, in addition to many others, have subsequently been borne out by observation. Not only was Einstein’s theory correct in describing the phenomena that should arise, but the magnitude of the effects observed precisely matches general relativity’s predictions.
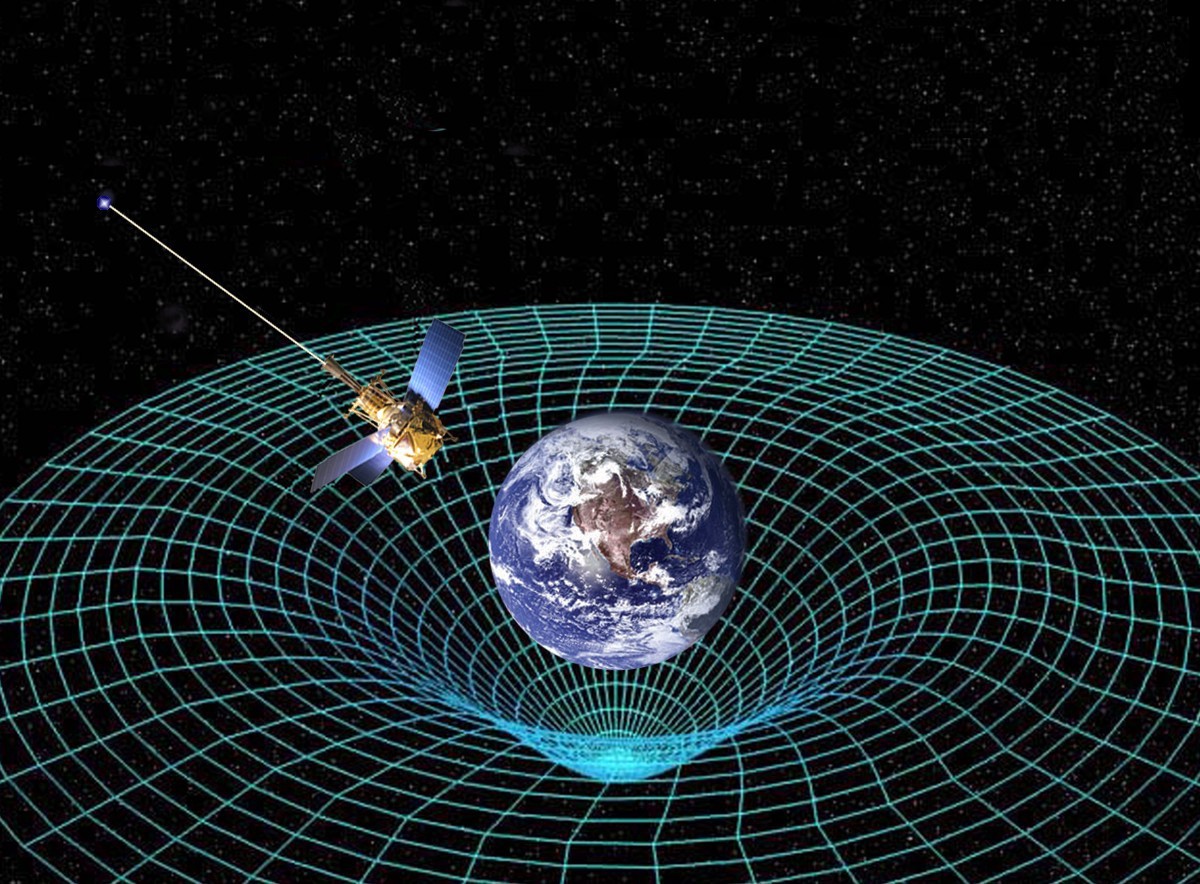
Dragging spacetime
All the way back in 1918, scientists Josef Lense and Hans Thirring calculated how a rotating body about one axis, such as a gyroscope, should precess by an “extra” amount due to the presence of a nearby large, rotating mass that distorts the surrounding spacetime, such as the spinning Earth. This effect, known as Lense-Thirring precession, would go untested for many decades, until a combination of astrophysical tests and direct experimental tests became precise enough to probe the effect predicted within Einstein’s theory.
On the experimental front, the Gravity Probe B experiment confirmed the expected geodetic effect to within about 0.3% and the effects of frame dragging to within around ~15% between 2004-2011.
Astrophysically, the direction in which an astrophysical jet around a black hole points can rapidly change due to a reorientation of the accretion disk, which can be caused by the Lense-Thirring effect; this was observed in 2019 around the black hole X-ray binary V404 Cygni. Pulsar timing in a system where the companion is a close-by, rapidly rotating white dwarf has also revealed the Lense-Thirring effect, and future observations of stars in the galactic center as well as by the Juno spacecraft, presently in orbit around Jupiter, are poised to provide further validation of this prediction.

Gravitational waves
Perhaps the longest-awaited prediction to be confirmed was the existence of gravitational waves: predicted as a consequence of general relativity almost immediately (and by Einstein himself, no less) but not observed for a century, until the first detection of merging black holes by LIGO in 2015. Although gravitational waves were expected to exist based on indirect evidence seen in the decay of a binary pulsar system, this novel type of direct detection was unprecedented. Today, well over 100 candidate gravitational wave events have been seen, and gravitational wave astronomy is a young and growing field that allows us to explore the Universe gravitationally, without relying on light of any type.
Many other predictions of general relativity have been teased out of the theory and put to the test, including the Shapiro time delay, gravitational lensing, precise tests of the equivalence principle using lunar laser ranging and torsion balance experiments, tests of gravitational redshift and gravitational time dilation used in global positioning systems, and small-scale tests of the gravitational law down to scales as small as 55 microns. Wherever we’ve been able to tease physically relevant predictions out of general relativity and put it to the test, Einstein’s theory has passed with flying colors.
At a fundamental level, we have two classes of physical theories: the quantum field theories that describe the strong, weak, and electromagnetic forces, and Einstein’s general relativity that describes the gravitational force. Even though it was put forth as far back as 1915 — before the discovery of the muon, quarks, the neutrino, or even the neutron — it remains undefeated as a physical theory, despite being perhaps the most challenged and scrutinized idea in all of scientific history. It’s the unparalleled success of general relativity, so thoroughly and for so long, that makes Einstein such a revered figure, and perhaps the greatest physicist of all time, even according to physicists.