Earliest Signal Ever: Scientists Find Relic Neutrinos From 1 Second After The Big Bang
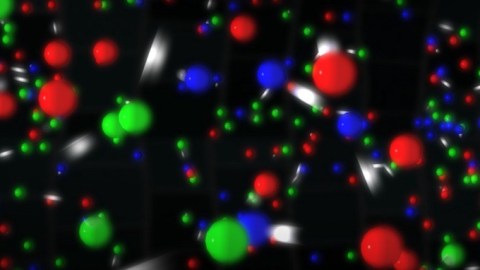
Before we formed stars, atoms, elements, or even got rid of our antimatter, the Big Bang made neutrinos. And we found them.
The idea of the Big Bang has captivated the imagination of humanity since it was first proposed. If the Universe is expanding today, then we can extrapolate back, earlier and earlier, to when it was smaller, younger, denser, and hotter. You could go back as far as you can imagine: before humans, before the stars, before there were even neutral atoms. At the earliest times of all, you’d make all the particles and antiparticles possible, including the fundamental ones that we cannot create at our low energies today.
If this were true, there would be an early signal left over from when the Universe was just one second old: neutrinos and antineutrinos. Known as the cosmic neutrino background (CNB), it was theorized generations ago, but was dismissed as undetectable. Until now. A very clever team of scientists has just found a way to see it. The data is in, and the results are incontrovertible: the cosmic neutrino background is real, and agrees with the Big Bang.
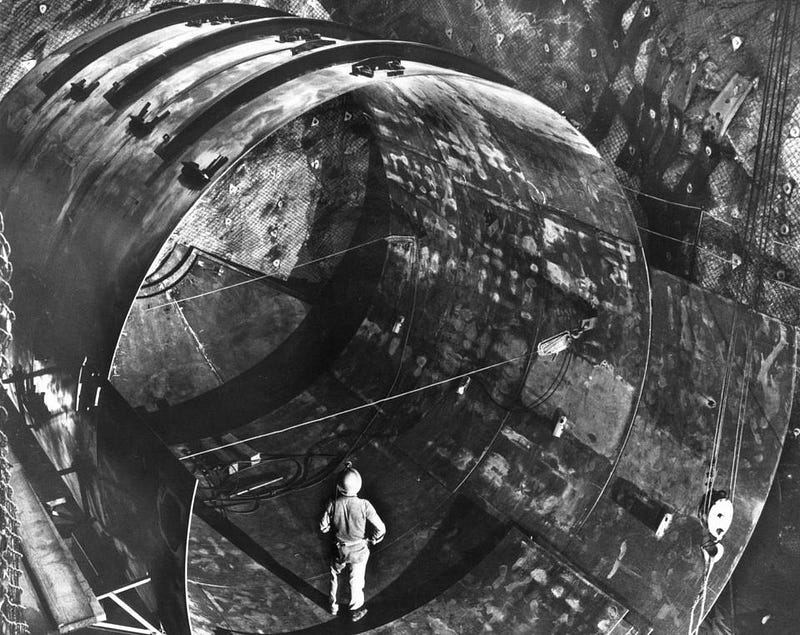
Neutrinos are some of the most surprising and elusive particles in the Universe. They were predicted in 1930 to explain radioactive decays; their name means “tiny, neutral one” to explain the fact that they must carry energy and momentum, but cannot have charge and must be incredibly low in mass. It wasn’t until we developed nuclear reactors that we were able to first detect their presence, a feat that wasn’t accomplished until 1956.
But neutrinos are real, and they’re fundamental, just like electrons or quarks are. They interact only through the weak and gravitational forces, so they neither absorb or emit light. At high energies, like those achieved in the earliest stages of the hot Big Bang, the weak interactions are much stronger. That’s where we can create enormous amounts of both neutrinos and their antimatter counterparts, antineutrinos.
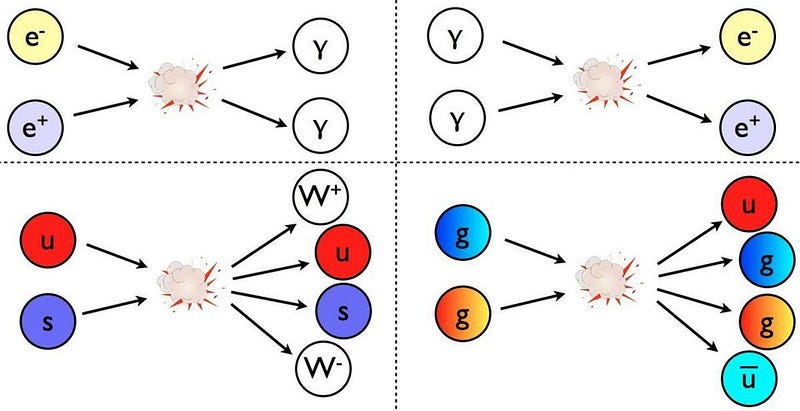
Whenever particles smash together, they can spontaneously create new particle/antiparticle pairs, so long as there’s enough energy present. When we rewind the clock on the Universe to extremely early times, we have enough energy to create all the particles and antiparticles we know of: all the quarks, leptons, and bosons that can exist. When the Universe cools, particles and antiparticles annihilate away, unstable particles decay, and you no longer have enough energy to create new particles.
This leaves us, later on, with only a small, leftover amount of matter compared to the bath of radiation that remains. That radiation isn’t just made of photons (particles of light), however. The neutrinos and antineutrinos stop interacting when the Universe is just one second old, and since they can’t decay into anything, they should remain until the present day.
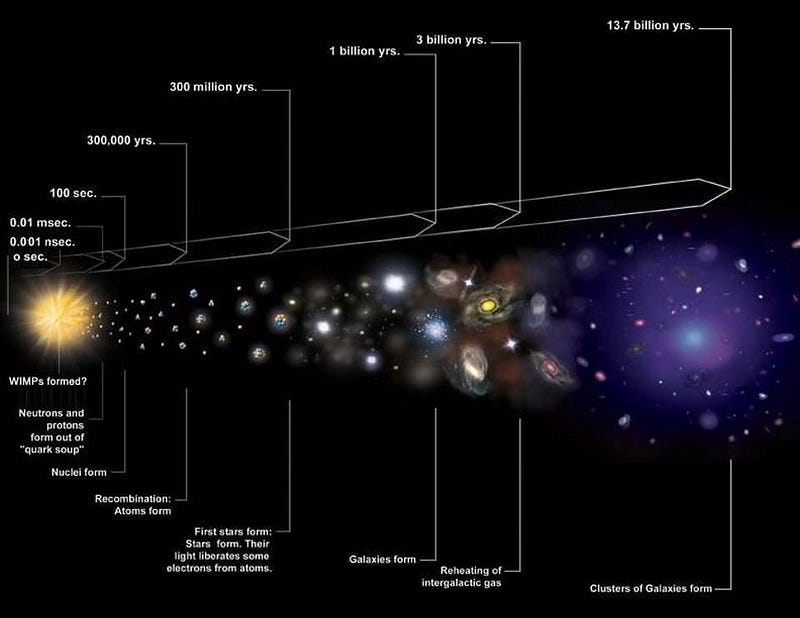
As the Universe evolves, all sorts of fascinating things happen. The quarks form protons and neutrons, which fuse into the first atomic nuclei, which gravitate together, which form neutral atoms, which then clump and cluster together into stars and galaxies. The leftover photons, meanwhile, smash into all the charged particles for hundreds of thousands of years, pushing on the normal matter and exerting pressure, and then stream freely through space once the neutral atoms form. That leftover radiation still exists today as the cosmic microwave background (CMB).
The neutrinos and antineutrinos, on the other hand, never had those interactions. They didn’t smash into charged particles. They simply streamed freely through the Universe at nearly the speed of light, and then slowed down as the Universe expanded. Owing to their tiny but non-zero masses, they should still exist today, falling into galaxies and clusters of galaxies at late times.
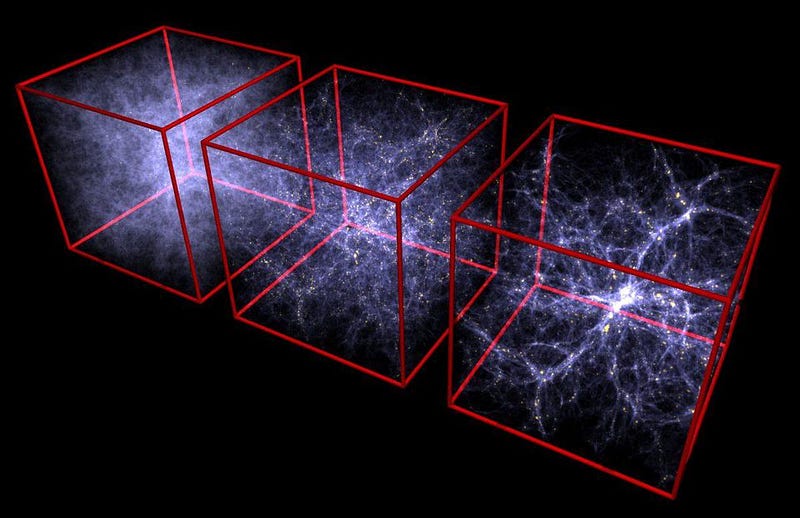
This cosmic neutrino background (CNB) has been theorized to exist for practically as long as the Big Bang has been around, but has never been directly detected. Because neutrinos have such a tiny cross-section with other particles, we generally need them to be at very high energies in order to see them. The energy imparted to each neutrino leftover from the Big Bang corresponds to only 168 micro-electron-volts (μeV) today, while the neutrinos we can measure have many billions of times as much energy. No proposed experiments are theoretically capable of seeing them unless some exotic physics is at play.
But there are two ways to see them indirectly: from their effects on the CMB and on the large-scale structure of the Universe. The seeds for both the CMB and the large-scale structure we see today were planted early on, when the neutrinos were more energetic and more important. In fact, when the CMB was emitted, neutrinos were an important fraction of the total energy in the Universe!
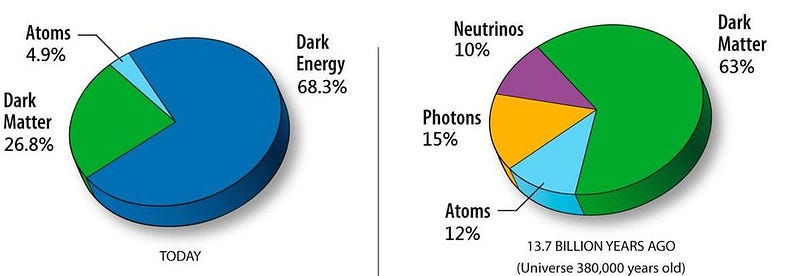
Because they behave like radiation does at very early times, they will smooth out the seeds of large-scale structure by streaming out of it. You can imagine the young Universe as being filled with tiny clumps of matter: overdense regions where there’s just slightly more mass than average in them. If it weren’t for radiation, these clumps would just start to grow under the influence of gravity. An overdense region would attract more mass, and would grow and grow unchecked, in a runaway fashion.
But radiation has energy, too, and always moves through empty space at the speed of light. As your mass clumps grow, the radiation that’s in them preferentially streams out of them, stopping their growth and causing them to shrink again. This is why there’s a particular pattern of peaks and valleys in both the CMB and in the large-scale structure of the Universe.
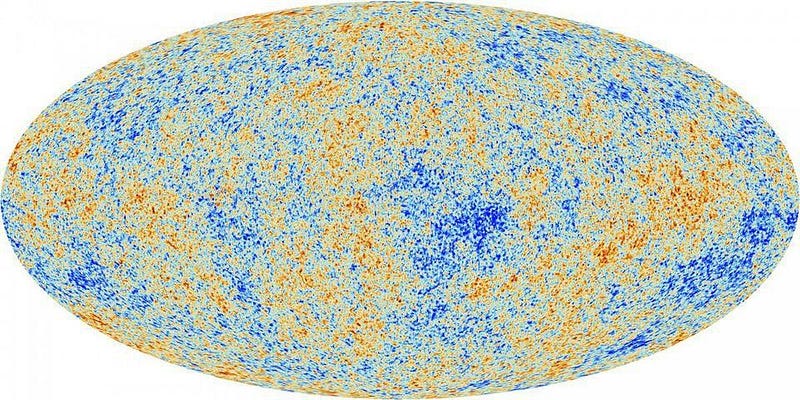
These neutrinos, if the cosmic neutrino background (CNB) is real, will affect both the CMB and the Universe’s large-scale structure.
The effects on the CMB will be subtle, but measurable. The pattern of peaks and valleys will be stretched out and moved to larger scales — albeit extremely slightly — by the presence of neutrinos. In terms of what can be observed, the peaks and valleys will have their phases shifted by a measurable amount that depends on both the number of neutrinos that exist and the temperature (or energy) of those neutrinos at early times.
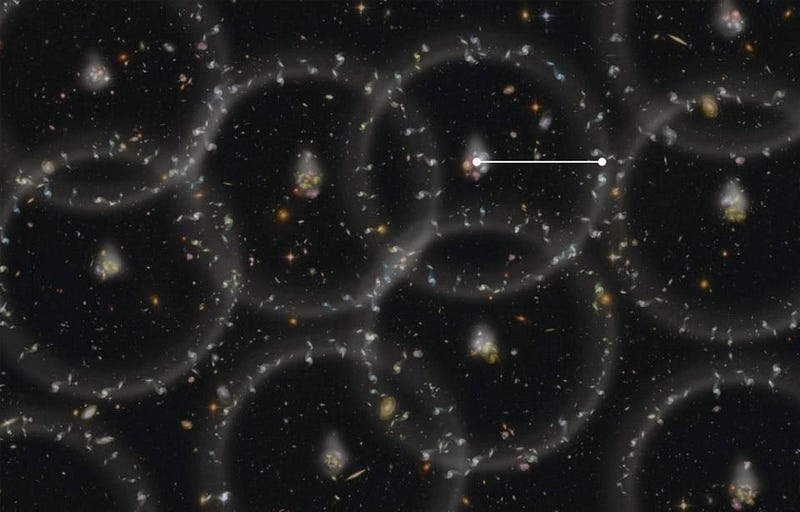
Meanwhile, the effects on the large-scale structure will also be subtle, but theoretically measurable as well. There are scales today on which we’re more (or less) statistically likely to find another galaxy than average, depending on how far away we’re looking from a particular galaxy and how much the Universe has expanded.
Although the effect is small, there will be a shift in that distance scale and the particular shape of the curve owing to the neutrinos, which stream out to slightly larger distances, ahead of the rest of the matter. These changes are dependent on how many neutrinos there are, what their energy is, and how they behave in the early Universe. The CNB may not be directly detectable today, but its indirect effects on two observables — the CMB and the large-scale structure of the Universe — should be able to be detected even now.
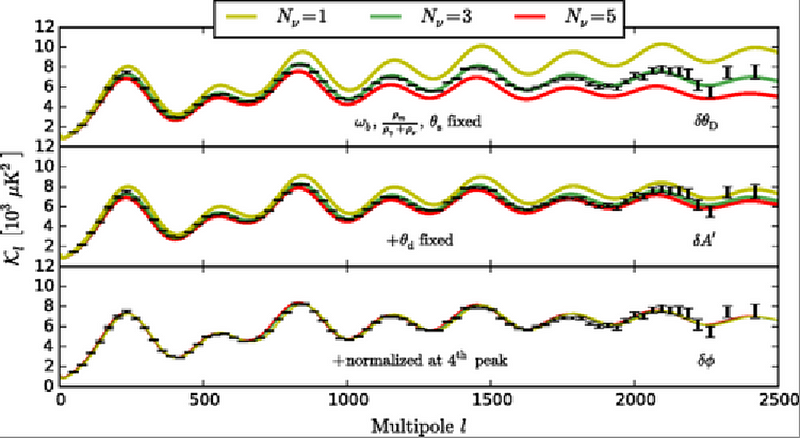
Remarkably, the effects of these early, relic neutrinos were detected in the CMB back in 2015, and were consistent with there being three species of light neutrino, consistent with the electron, muon, and tau species we’ve directly detected today. By looking at the polarization data from the Planck satellite, as announced at the 2016 AAS meeting, the team was also able to determine the energy of the CNB: 169 μeV, with an uncertainty of ±2 μeV.
It was a remarkable confirmation of the Big Bang’s predictions for the CNB, but everyone was still waiting for the data from the large-scale structure.
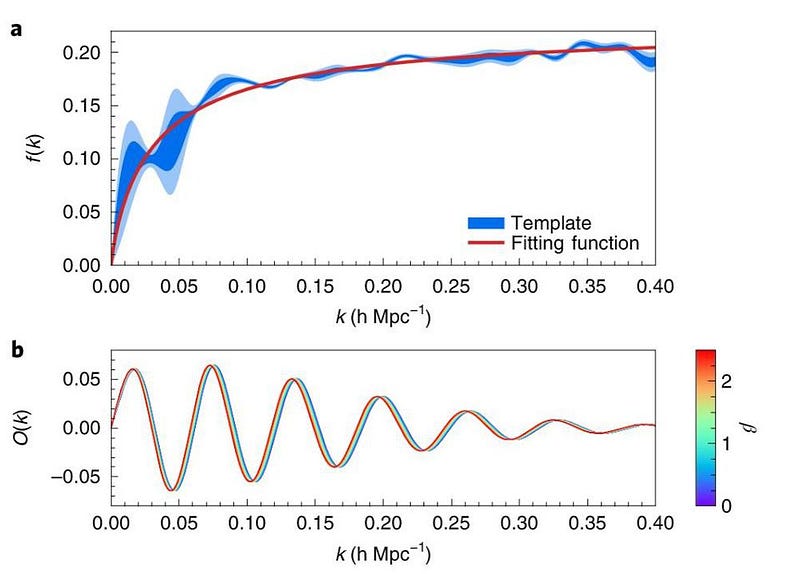
Our best measurements of the likelihood of finding a nearby galaxy dependent on distance scales in the Universe comes from enormous galaxy surveys that cover wide fields of view and extend to extremely large redshifts and distances. The features that we see as peaks and valleys in terms of your likelihood of finding a galaxy at a particular distance are known as baryon acoustic oscillations, and the best data set we have for measuring them comes from the Sloan Digital Sky Survey (SDSS).
As reported in Nature this week (a preprint from 2018 is available here), we now have the first robust measurement of the phase shifts due to neutrinos. Although the results don’t really lend themselves to a stunning visual presentation, what you need to know is that there are two parameters that they vary to see how good their results are: α and β. For the Big Bang’s predictions of the CNB, α and β should both equal 1, exactly.
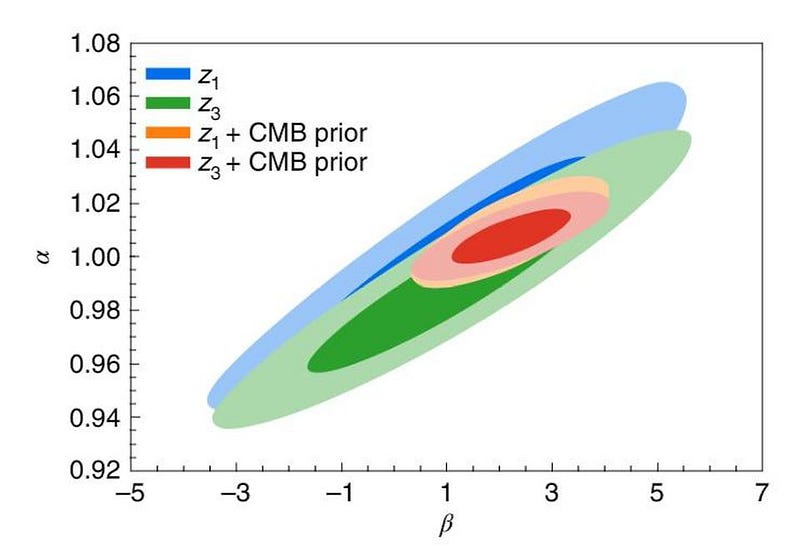
As you can see, the constraint on α is very good; the constraint on β isn’t as good. However, it’s good enough that we can rule out β=0, which is what we would get if there were no cosmic neutrino background. Even with our very first positive results, we can establish that, for the first time, the cosmic neutrino background has been detected in the large-scale structure of the Universe. A robust signal, created just 1 second after the Big Bang, has been definitively seen and measured.
This first measurement isn’t the end, but merely the beginning, of probing the CNB. While there are plans to improve what is known from the CMB as far as measuring the presence of neutrinos goes, the large-scale structure of the Universe is just getting started. The Sloan Digital Sky Survey is about to be superseded by newer, more powerful telescopes over the next decade, revealing parts of the Universe that remain invisible to us today.
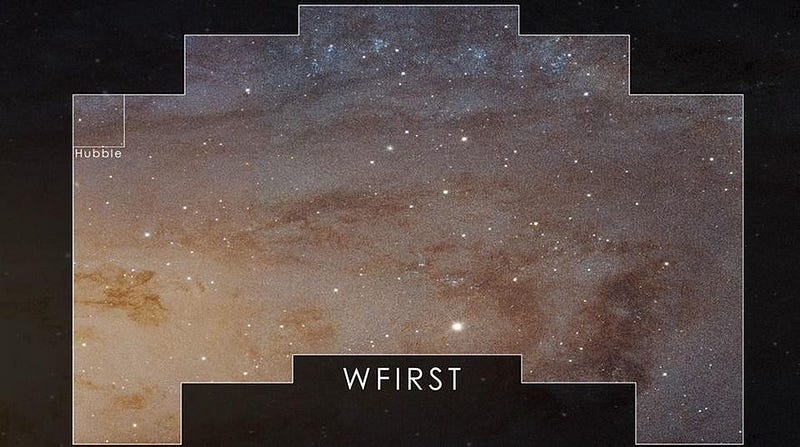
Future surveys to be conducted with upcoming telescopes and observatories, including DESI, Euclid, WFIRST and the LSST, will all improve on these results dramatically. The energy that each neutrino had at these early times corresponds to a temperature, today, of just 1.95 K, which makes it even colder than the leftover glow from the Big Bang.
Now that we’ve not only detected the CNB, but confirmed its existence, it’s time to learn everything we can. It wasn’t clear, even with all the data we’ve collected so far, that we’d be able to identify this signal when put up against all the other sources of uncertainty (like nonlinear evolution), but the effect clearly shines through. Most importantly, it’s a spectacular confirmation of the Big Bang, which once again demonstrates that it’s the only viable game in town.
Ethan Siegel is the author of Beyond the Galaxy and Treknology. You can pre-order his third book, currently in development: the Encyclopaedia Cosmologica.