Ask Ethan: Can we detect dark matter if it’s truly collisionless?
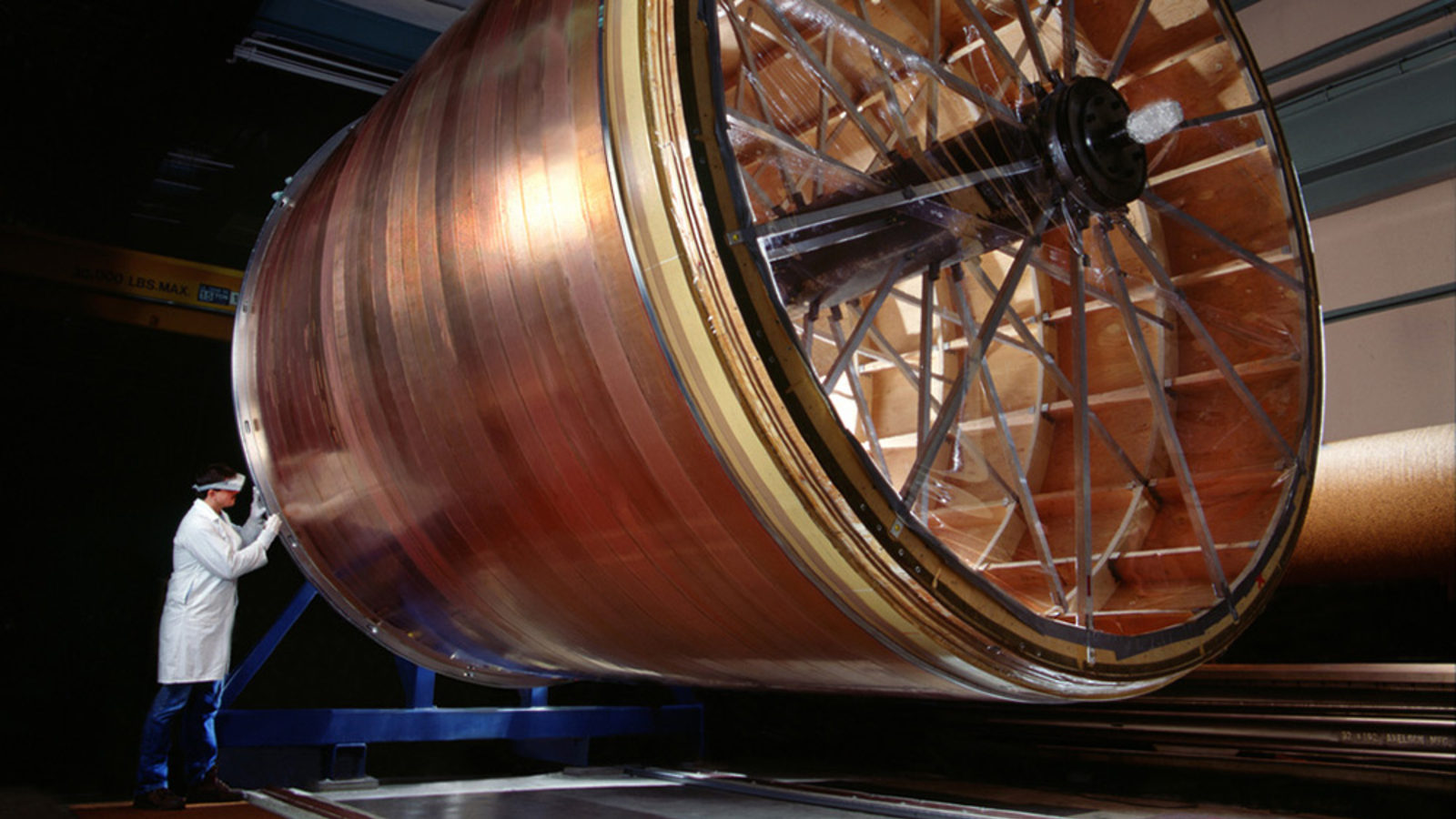
- Dark matter is one of the most mysterious substances in the Universe, outmassing normal matter by a 5:1 margin but showing no evidence of interacting otherwise, either with normal matter or itself.
- There are a great many direct detection efforts underway, but all have proven fruitless so far, including nuclear recoil experiments and annihilation experiments, yielding only limits on dark matter’s properties.
- Many will not be convinced of dark matter’s existence unless direct detection efforts succeed, but there’s no guarantee that our current approaches will ever reveal it. What if dark matter is truly collisionless?
One of the great mysteries in all the Universe is the question of what, exactly, makes it all up? The science of experimental particle physics revealed an incredible part of that story, discovering that everything that makes up ourselves and all the objects we can directly interact with is represented within the Standard Model: quarks, charged leptons, neutrinos, photons, gluons, and even heavy, unstable bosons like the W, Z, and Higgs. Yet all of this — all that we see, know, and detect — makes up only about 5% of the total energy budget of the Universe. A whopping ~27%, meanwhile, is made of a mysterious substance that behaves like a massive particle, known as dark matter, and the remaining ~68% is known as dark energy, which behaves as though it’s a form of energy inherent to space itself.
While there’s an overwhelming suite of observational evidence indicating dark matter’s existence and influence on cosmic structures, from galaxies to galaxy groups, clusters, filaments, and the large-scale cosmic web, all direct detection experiments to date have only turned up null results. This lack of direct detection leads many to doubt its existence, for very understandable reasons. Is it possible that all direct detection efforts are doomed to ultimate failure? That’s what Dr. Able Lawrence wants to know, writing in to ask:
“If [dark matter] is collisionless, even with itself, how will you detect it using detectors that look for collisions?”
It’s a great and troubling question, and one we must reckon with very seriously. Here’s what the science has to say about it.

Observationally, there’s an overwhelming amount of evidence that indicates the presence of a species of energy that has existed from very early times in the Universe’s history that gravitates like the matter we’re familiar with does, but that shows no evidence for interacting in any other way, either with itself or with any of the known particles of the Standard Model. We see evidence for the presence of this unfamiliar material, which serves as a source of gravity, across a wide variety of cosmic probes. It shows up:
- when we weigh individual galaxies,
- when we weigh pairs of galaxies that are interacting,
- when we measure the gravitational lensing effects of galaxies, galaxy groups, or clusters of galaxies,
- when we tally up the total amount of normal matter in stars, gas, dust, plasma, and black holes, and compare it with the total mass that we see in a variety of cosmic objects,
- when we examine the pattern of fluctuations present in the CMB, the leftover glow from the Big Bang,
- when we examine the clustering patterns imprinted in the cosmic web,
- and when we measure a feature known as baryon acoustic oscillations, imprinted in galaxy-galaxy correlations across hundreds of millions of light-years.
All of these independently measurable lines of evidence indicate that the normal matter we see is wholly insufficient to explain the Universe we observe on its own. Something else, in addition, must be present.
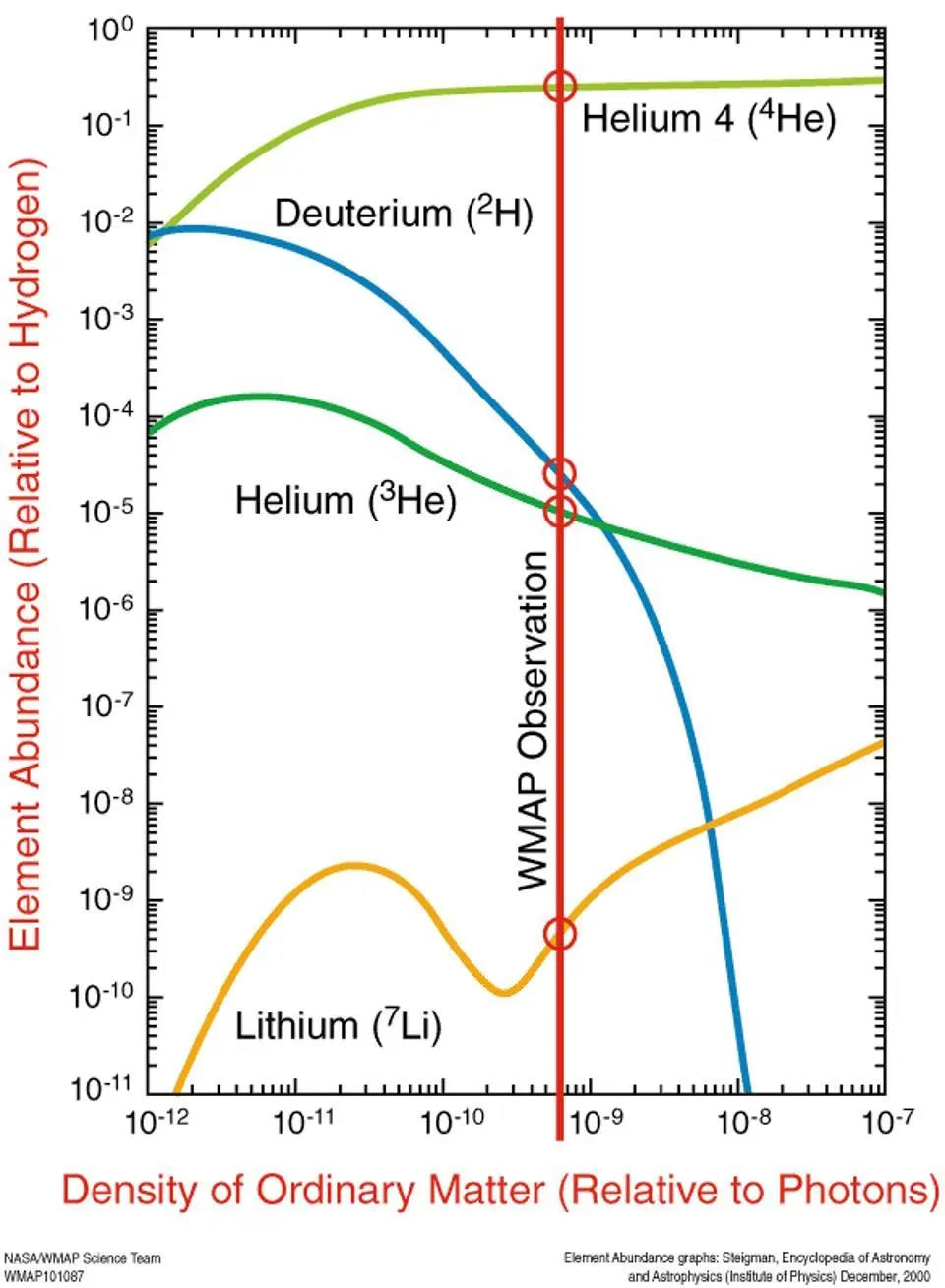
Another strong, but often overlooked, piece of evidence is shown above: the abundance of the light elements and isotopes, which were forged long before the first star in the Universe ever ignited. Early on, in the crucible of the Big Bang, temperatures were high enough and densities were great enough that nuclear reactions could proceed, where protons and neutrons would undergo fusion reactions, creating a variety of light elements and their isotopes in some non-negligible abundance. This included the forging of:
- deuterium (one proton and one neutron),
- tritium (one proton and two neutrons, which will decay to helium-3),
- helium-3 (two protons and one neutron),
- helium-4 (two protons and two neutrons),
- lithium-7 (three protons and four neutrons),
- beryllium-7 (four protons and three neutrons, which will decay to lithium-7),
and tiny amounts of even heavier elements, like beryllium-9 and beyond.
The nuclear physics that took place in the very early Universe — known today as Big Bang Nucleosynthesis — is extraordinary because all of the reactions that occur, leaving behind a specific abundance of these various elements and isotopes relative to one another, is dependent only on one cosmological parameter: the baryon-to-photon ratio. We’ve since measured that value exquisitely, and found that it’s about 6 × 10-10, meaning there’s about 1 baryon (i.e., proton or neutron) for every 1.7 billion photons in the Universe. That corresponds to a matter density that’s about 5% of the overall density of the Universe, but measurements of the total amount of matter in the Universe, from galaxy clusters and gravitational lensing observations, for instance, reveal a figure that’s more like ~30% of the Universe’s total energy density.
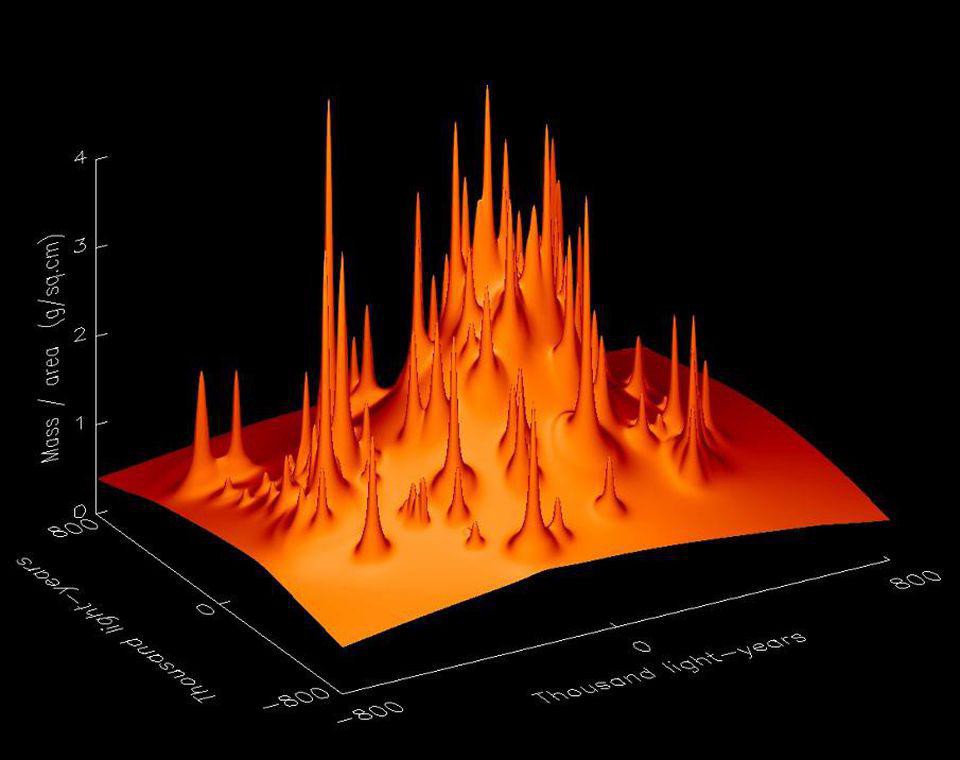
All of these lines of evidence, when put together, clearly indicate the presence of some type of mass in the Universe that isn’t part of our Standard Model. Moreover, that mass has to have specific properties that are highly constrained by cosmic observables.
- It must have existed from very early times, as its signals are thoroughly imprinted in the CMB, which was emitted just 380,000 years after the Big Bang.
- It must have been moving slowly compared to the speed of light, or must have been what cosmologists call “cold,” even from the moment of its birth, implying that it was either created non-relativistically or, if it was created thermally, it was heavy enough that at no point in cosmic history was it moving fast relative to the speed of light.
- It must not have annihilated away with itself, must not have clumped together like normal matter does, and must not have interacted electromagnetically, through the strong force, or through the weak interactions in any appreciable, measurable way even from early times.
For these reasons, what was originally referred to as the “missing mass” problem became known as the “dark matter” problem, as it appeared that the Universe must contain some type of massive species of energy that gravitates, but that doesn’t emit or absorb light, and doesn’t interact or collide with normal matter or itself in any detectable way.
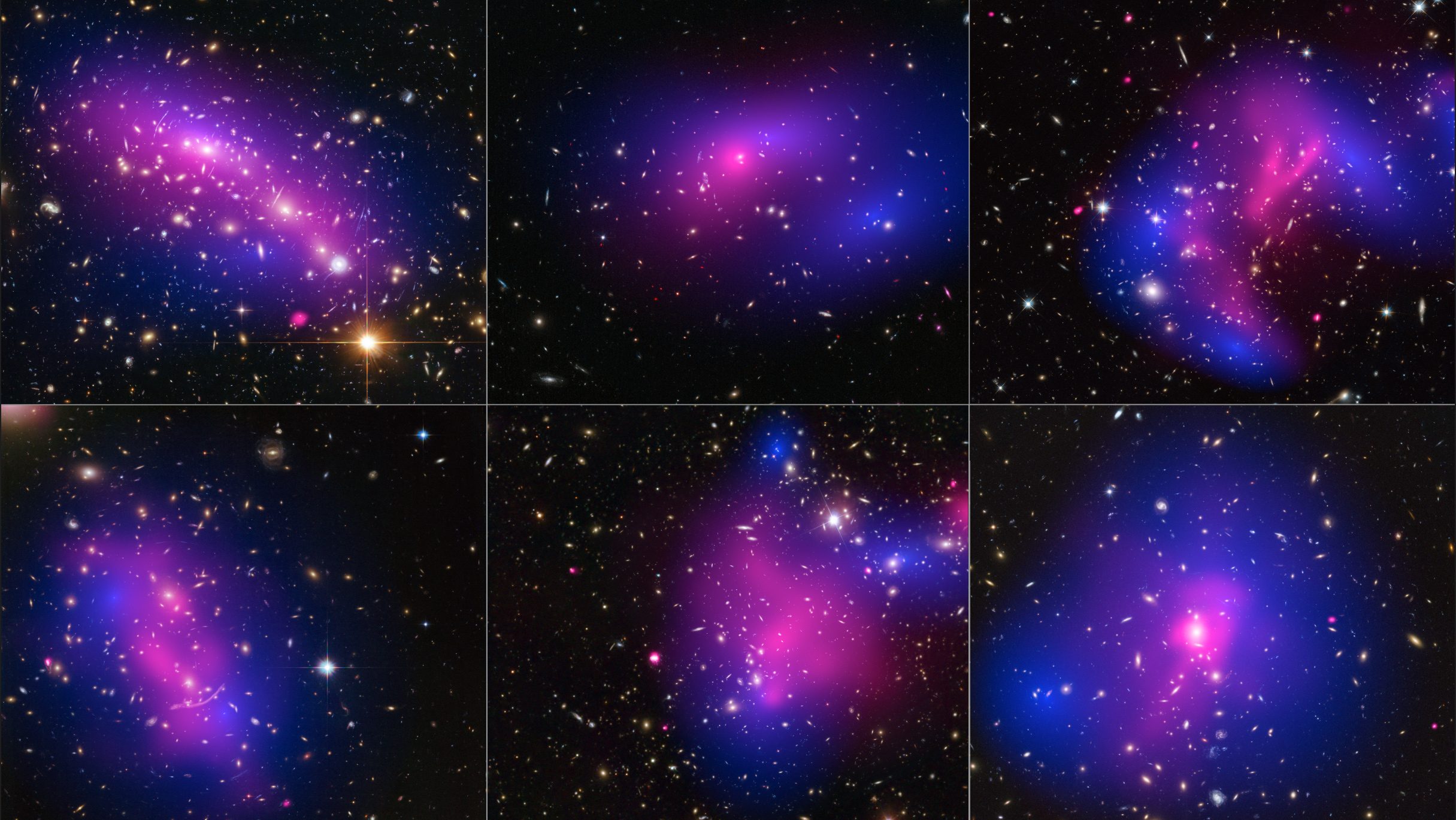
Beginning in the mid-2000s, we started to even detect clear signatures that some type of massive signature must be present within galaxies and galaxy clusters, as colliding systems began to reveal a clear separation between the normal matter, which heats up and emits X-rays, and the total mass, which is revealed from gravitational lensing signals. The evidence from the full suite of evidence strongly indicates the presence of a massive new species of energy, which experiences the force of gravity and clumps together on cosmic scales, but which doesn’t “splat” together, interact with light, or make bound structures with either normal matter or itself in any discernible way.
So what could this dark matter be, and how could we detect it? There are two main ideas that have been pursued since the latter half of the 20th century.
- Dark matter could be WIMP-like, meaning that there could have been a massive, heavy particle beyond the Standard Model that was once produced, in great abundances, in the early stages of the hot Big Bang. As the Universe cooled, some species of massive, relic particles persisted, moving slowly compared to the speed of light, and it still persists today. Although rare, interactions and collisions with Standard Model particles could still occur, since that’s how these particles, at very high energies, were created in the first place.
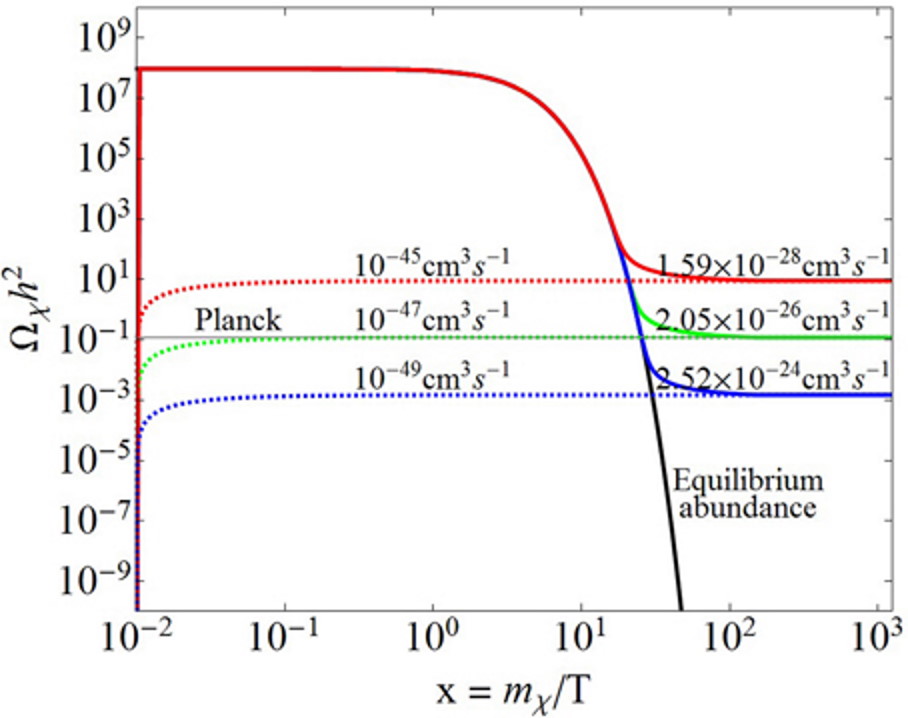
- Dark matter could also be axion-like, in the sense that it could be made of particles — of any mass, not just heavy ones — that were pulled out of the quantum vacuum during a phase transition in the early Universe, but that were born cold: with practically no kinetic energy at all. The hypothesized Peccei-Quinn symmetry, and the breaking of that symmetry, was the origin of the axions-as-dark-matter idea, and of this mechanism. Even though that idea in its original incarnation has now been ruled out, the search for axions and axion-like particles continues.
The way you search for WIMP-like particles is to build experiments that look for nuclear recoils, as there should be some non-zero cross-section, at least at some high energy (and presumably, at lower energies too), between whatever dark matter is and at least one of the Standard Model particles.
Complementarily, the way you search for axion-like particles is to build an electromagnetic cavity that’s capable of exploiting a theoretical coupling between axions (or axion-like particles) and photons. At low temperatures and when tuned to specific frequencies, any dark matter particles that happen to be passing through the detectors, dependent on their mass and cross-section, could be converted into photons, and detecting those photons would then be a telltale signature of dark matter.

But here’s the thing: dark matter doesn’t have to be a thermal relic that arose from interactions with Standard Model particles, and it doesn’t have to be a “pulled from the vacuum” relic that will couple to the photon (or any other Standard Model particle). It could be:
- a heavy, exotic, right-handed neutrino that was created at the start of the hot Big Bang and never interacts with anything in the Standard Model,
- an ultra-heavy WIMPzilla particle that was created early on through purely gravitational processes, and will never interact with any Standard Model particle through any force,
- or something more general that arose from a severely out-of-equilibrium condition, which wouldn’t necessarily interact with any of the Standard Model particles or fields through the strong, electromagnetic, or weak interactions.
If any of these are the case, then all our current classes of experiment will do is continue to push down, to lower and lower boundaries, the cross-section of whatever dark matter is in a certain mass range below a certain limit. This is an incredible feat of experimental physics, but it isn’t ever going to reveal dark matter’s nature, directly, if this is the case.

A lot of people think that because we talk about dark matter as possibly being made up of WIMPs, where WIMP stands for Weakly Interacting Massive Particle, that we fully expect it to interact through the weak interaction. This is not true! There is a scenario known as the “WIMP miracle,” where if you make a thermal relic in, say, supersymmetric or similar scenarios, the cross-section you need to produce the right abundance of massive particles to match observations just happens to match the predicted cross-sections that would proceed through the weak interactions. However, as LHC data is more and more convincingly showing, the parameter space for the WIMP miracle scenario is almost entirely excluded. The WIMP miracle, except among the most fervent supersymmetry believers, is almost totally dead.
Instead, I think it’s worth recognizing that what we would have once considered a nightmare scenario for dark matter — the possibility that it has no interactions to observe other than gravitational ones — might in fact be the scenario that nature has presented us with. The theoretical motivation for completely collisionless dark matter is very strong, as there are:
- no signals indicating collisions with atomic nuclei,
- no signals indicating conversion to photons,
- and no signals indicating a self-interaction or self-annihilation cross-section,
and yet, the observational evidence indicating dark matter’s presence is overwhelming.
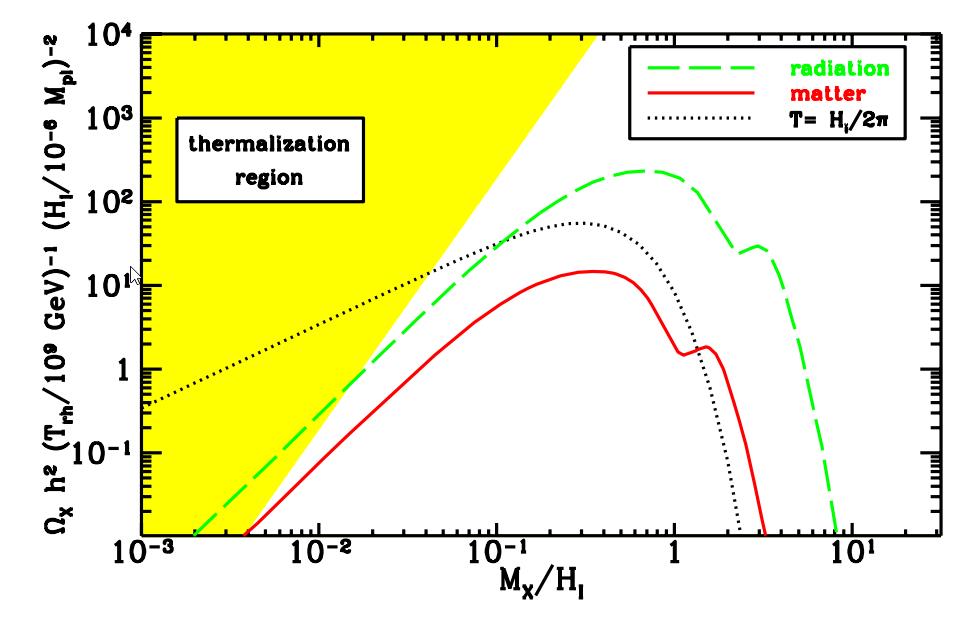
This is not a flaw or a fault in our techniques; this is a limitation imposed by what the Universe gives us to work with. It is only normal, Standard Model particles that we know how to interact with. If we think more generically, any species of energy that strikes a particle — a quark, a gluon, an electron, etc. — is going to impart energy and momentum to it whenever it occurs. We can build enormous detectors around collections of matter, things made of quarks, gluons, and electrons, and look for any of the downstream signatures that are generated by such a collision.
The goal shouldn’t be “to find dark matter,” as that’s assuming we know what nature is up to, and that is not a thing we’re able to know until we find out. The goal should be to interrogate nature as comprehensively as possible, and to put as many well-motivated (or even not-ruled-out) scenarios to the critical test as possible. The push to make experiments that take:
- larger, more pristine samples of material,
- and observe them for longer periods of time,
- with well-accounted-for backgrounds and ever-tinier uncertainties and errors,
is literally the best we can do with the Universe we have. While there are many prominent voices who fail to see the scientific value in this, the only alternative is to throw our hands up in the air and give up before even making the attempt.
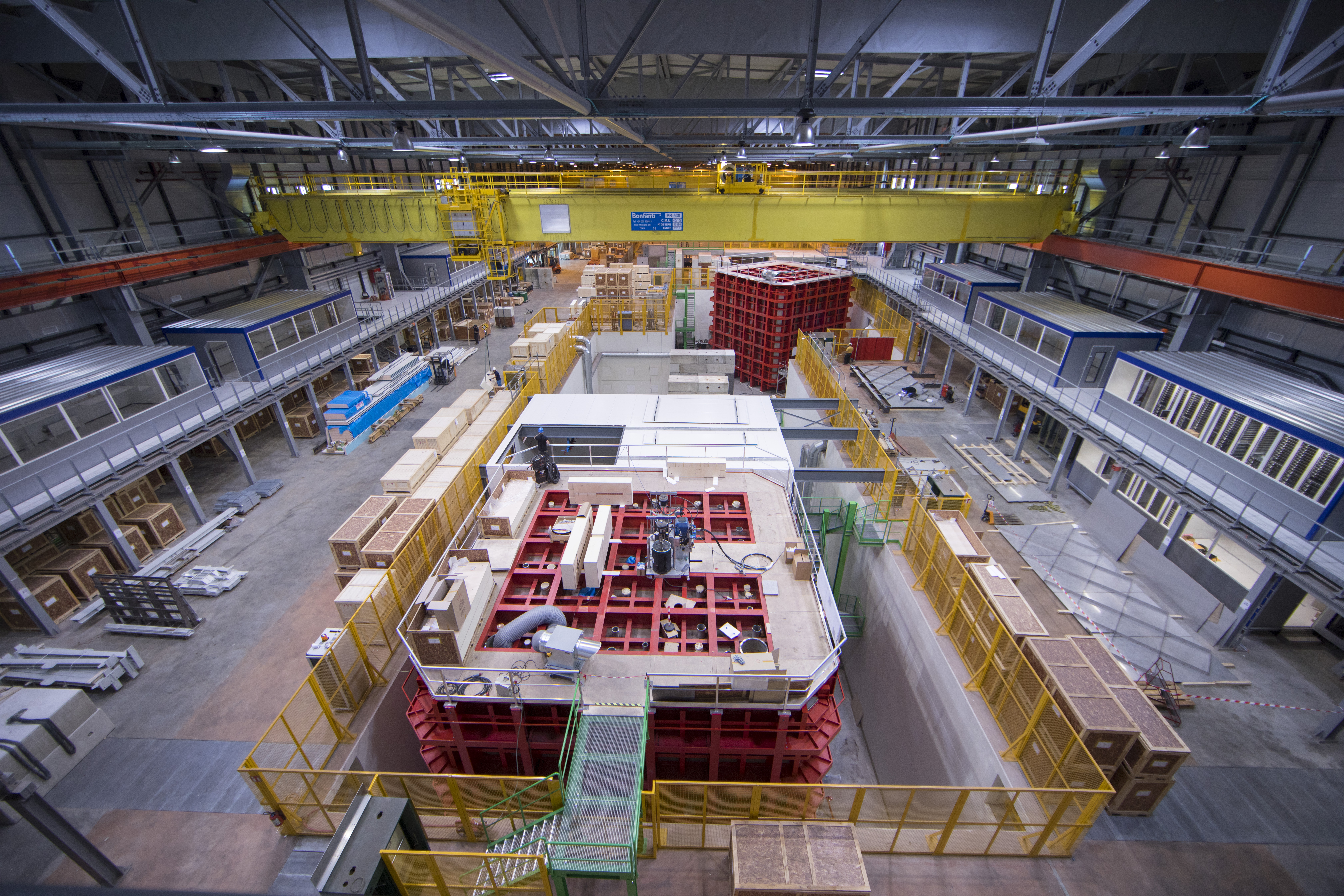
As I write this in the summer of 2024, when we witness the best athletes in the world giving it their all in competition, “giving up” seems like the most cowardly, unambitious approach possible. Sure, even the best experiments we’re capable of conducting have inherent limitations to them, and only if certain assumptions about the collision-and-interaction properties of dark matter are true will any of our current techniques be able to yield a positive detection.
That doesn’t mean these experiments are valueless; it rather underscores the importance of conducting them. Dark matter’s nature is unknown, and if we want to better know its nature, these are not only the best experiments we can conduct, these are the only types of experiments we know how to perform that have a chance of better constraining — or, if we’re fortunate, revealing — dark matter’s true properties.
Whenever we’re in the realm of the unknown, venturing beyond the frontiers of present-day knowledge, we are compelled to both maximize our discovery potential while simultaneously making sure we don’t fall victim to wishful thinking, and finding something phantasmal that conforms to our hopes and expectations. This approach to fundamental science has brought us to where we are today, and continues to help us probe the nature of reality at an elementary level to better precision than ever before. Yes, if dark matter is truly collisionless, these experiments we’re conducting and planning and building will not reveal its nature. But science is a cumulative endeavor, and every step we take along the path has valuable lessons to teach us, if only we’re willing to learn from what nature reveals about itself.
Send in your Ask Ethan questions to startswithabang at gmail dot com!