Complexity is beautiful, too
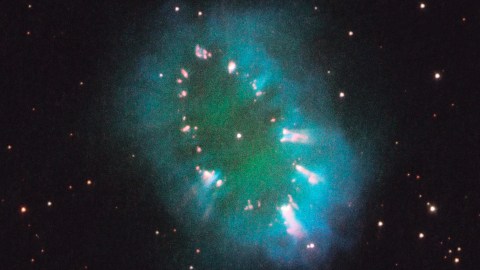
We hear a lot these days about beauty and science.
In the domains of fundamental physics—the birth of the universe, the nature of matter—physicists often fall in love with problems that have beautiful solutions. Here beauty tends to mean descriptive economy and simplicity mixed with explanatory richness. But what about problems that are not “fundamental”? What about the really complex stuff, the imminently more messy problems involving lots of different kinds of physics happening in different ways at the same time?
In that case, I will argue, problems aren’t “beautiful,” but they can be very, very tasty.
To illustrate what I mean, let me introduce you to the case of binary stars and what’s called common envelope evolution.
More than half of the stars in the night sky come with siblings. Instead of evolving through their birth, middle age, and death alone, they live with one or more companion stars. “Binary stars,” where two stars evolve together, are the most common form of multiple star system. If the stars orbit each other at a great distance then they run through their evolution barely noticing each other’s presence. They mature and die in almost the same way as single stars. But if the stars were born relatively close to each other, then their individual stories are intertwined, especially as they reach the end of their lives. For the (astro)physicist, that is where the definition of tastiness begins.
It took a long time for scientists to understand the evolution of single stars. Born when a cloud of interstellar gas collapses on itself, stars are powered by nuclear fusion reactions in their core. The energy (light) released in those reactions must squirm its way out the surface, and in doing so sets the structure of a star’s onion-like layers.
Luckily, single stars are basically spheres, helping to simplify calculations to understand their evolution. That’s why we can say with confidence that we understand most of the evolution of normal single stars.
But binary stars are a whole different story. Because they are constantly swinging around each other, two stars in a tight orbit distort each other in ways that are complex and very difficult to calculate. Even after years of trying, we astrophysicists still have a boatload of unanswered questions about binary star evolution. Among these questions, the short, violent phase called common envelope evolution reigns supreme.
When stars like the sun reach the end of their lives, they swell to sizes larger than Earth’s orbit. This “giant” phase gets complicated when there’s a companion star close enough to get swallowed by the expanding outer layers of the bloated giant. Dragged by friction from the giant’s atmosphere, the companion quickly spirals inward, as its orbital energy is drained into heating the gas of the now “common envelope” of the two stars. In just a matter of days, the cores of the two stars will end up so close to each other that their orbit takes them only days to complete. The common envelope, containing many times the mass of the sun, will be blown into space forever—resulting in something like the necklace nebula shown at the top of this page.
This common envelope story is essential for astronomers because it’s almost the only way to explain Nature’s weirdest beasts. The black hole-black hole and neutron star-neutron star mergers which made headlines—confirming Einstein’s predictions about space and time—are probably the result of common envelopes.
In search of tastiness
So where’s the “tastiness” in all this? Well, here is the problem: We can’t make the common envelope process work. Understanding common envelope evolution requires the most sophisticated supercomputer simulations. That’s because the process is so violent and occurs so quickly that we need powerful computers to animate the governing math.
Over the last decade, a number of research groups—including my own—have managed to build and test sophisticated computer codes for simulating common envelope evolution. And while we have made really exciting progress, we have also failed—all of us. In particular, we have not yet been able to create a simulation that gets the two stars close enough to each other explain what we see on the sky. The inward spiral of the companion stalls far from the really short orbits we expect. As of this writing, nobody knows why.
But we do have ideas . . . a bunch of them. And that’s what makes the common envelope problem tasty. Those questions could be about:
- how radiation flows through the atmosphere of the wildly distorted gas of the swirling stars
- how atoms capture electrons as the expanding envelope gas cools
- light pushing on newly forming dust grains where the gas is cooling
- magnetic fields
Any one of these processes could be the key to solving the problem of common envelope evolution—or it could be all of them working together.
Unlike a new theory of space or time or matter, solving the common envelope problem is not about finding some simple, over-arching, elegant solution to a single set of equations. Instead, it’s about combining a number of very different kinds of physics (radiation, magnetic fields, fluid flows) and knowing to where to apply the right kind of intellectual pressure. The trick will be to find the right kinds of reasoning to cut through the mess of time-scales and length-scales on which the different physical processes work. We’ll begin with the simulations, which are both beautiful to watch and complex to interpret, and then we’ll have to unpack where and how the different kinds of physics are operating. Then, if we can think sharply enough, we might be able to pry away the messy crust of complexity and find an inner core of explanation.
So why is any of this “tasty”? Appreciating a problem like common envelope evolution, with its messy variety of physical processes, is a lot closer to appreciating a great meal than considering some beautiful abstract work of art. In considering great food, it’s the mix of flavors and spices (i.e., the different kinds of physics) that matter. It’s their subtle interplay that make the meal (the physics problem) interesting and worth pursuing. Rather than rejecting complexity as “ugly” for these kinds of problems, complexity is embraced just as it would be a well-prepared meal.
I raise this issue because it speaks to the different ways that scientists find meaning in their work. We often get the image of physicists contemplating the pure abstractions of space, time, and matter. But that is just one kind of physics—and a minority of the field. Taking on complexity is also the physicists’ job. And it’s in this very real-world domain that the aesthetics of taste may be a better metaphor than the beauty found in art.
The post Complexity Is Beautiful Too appeared first on ORBITER.