Why Don’t Dark Matter Simulations And Observations Match Up?
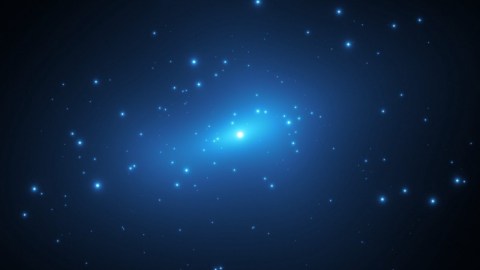
Could this finally be the clue we’ve hoped for in uncovering the truth about dark matter?
In the physical sciences, theory and observation are supposed to work hand-in-hand. Theorists work out the details of various ideas, yielding predictions for what the Universe should deliver under a variety of circumstances. Measurements and observations yield useful data about the Universe as it actually is, and those results can then be compared with various theoretical predictions. Ideally, one theory will emerge as successful, fitting the full suite of data available, while the alternatives fall away, disfavored by what the Universe tells us about itself.
For the past 40+ years, this has been the story of dark matter. By adding just one new ingredient to the Universe — a new species of cold, collisionless, massive particle — a whole suite of predictions could be extracted. Dark matter has implications for the Universe from small, irregular galaxies up to the enormous scales of the cosmic web or even the all-sky view of the cosmic microwave background. But a brand new study on the scales of galaxy clusters, where dark matter had previously been extremely successful, shows that simulations and observations don’t match up in an important way. Here’s the science of what’s really going on.
On the theory side, understanding what should happen in a galaxy cluster is a relatively simple concept. You start out with the Universe as we know it must have been early on: hot, dense, mostly uniform but with tiny imperfections (overdense and underdense regions), and filled with radiation, normal matter, and dark matter. As time goes on, the dark matter will gravitate but not collide with itself, normal matter, or radiation, while radiation and normal matter interact not only gravitationally but also through the other forces of the Universe.
Over time, a great cosmic web forms, with dense clumps of matter leading to galaxies forming along filamentary lines and rich galaxy clusters building up at the intersectional nexus of multiple filaments. While, on average, dark matter is expected to form an enormous, diffuse halo surrounding the normal matter, there will also be smaller “clumps” of dark matter that persist within the larger halo. The nature of dark matter determines the distribution of the various sizes, masses, and numbers of clumps within each halo.
Because dark matter only interacts gravitationally, it neither absorbs nor emits any light of its own. Technically, it doesn’t behave like something that we conventionally think of as dark; instead, dark matter acts as though it’s invisible. That might seem like it poses an insurmountable challenge to astronomers who are looking for its effects. After all, how can you hope to see something that’s invisible and doesn’t interact with matter or radiation directly?
The answer, perhaps surprisingly, is that you don’t need to be able to see dark matter in order to know its there. If we can predict what it’s distribution is — how much of it is located along any particular line-of-sight we look in — then we can calculate what its effects will be on all the light that passes through the region of space that it occupies. This is, perhaps, the most exciting feature of Einstein’s theory of gravity, General Relativity: matter and energy curve the fabric of space, and that curved space determines how matter and energy move.
Therefore, if we want to study dark matter, one of the most powerful things we can do is to look at very massive systems that require large amounts of dark matter to hold them together. Historically, some of the strongest observational evidence of dark matter has come from these rich galaxy clusters, as an additional gravitational effect well beyond what normal matter can account for is required to explain all that we observe.
This goes all the way back to the 1930s, when Fritz Zwicky was using the world’s largest telescope at the time, the 100-inch telescope atop Mt. Wilson — the same telescope Hubble used to discover the expanding Universe — to measure individual galaxies in the Coma Cluster. Because these galaxies are clustered together and we know how the law of gravity works, the speeds of the individual galaxies can be used to infer how massive the cluster must be.
Zwicky’s observations indicated that there wasn’t nearly enough normal matter present to keep the cluster bound together; if normal matter was all there was, these galaxies would be traveling far faster than “escape velocity,” meaning they’d fly off into space and the cluster would dissociate. Although his results weren’t taken seriously, they remain robust today. Without dark matter, the Coma Cluster (and many other galaxy clusters) wouldn’t have enough mass to hold their components together.
Over the years, many other cluster measurements support the existence of dark matter. Many clusters contain hot gas, which emits X-rays: we can measure how much “normal matter” is there and it’s only 11–15% of the required mass, leaving a need for dark matter beyond stars, gas and plasma. But the most important measurements are based on gravitational lensing, where the amount that light is curved, bent, magnified, and distorted reveals the total amount of mass present. In particular, when two galaxy clusters collide, we can literally see that the inferred mass and the observed location of the normal matter don’t match up.
Measurements like this have been around for a long time, indicating the overwhelming need for dark matter from a variety of independent observations. The Bullet Cluster, the first example of a colliding pair of galaxy clusters demonstrating the mismatch between the location of mass and the location of normal matter, is already 15 years old. But the decade-and-a-half that’s passed since then has given us more than just many examples of different systems that unambiguously illustrate these effects; they’ve also brought with them an increase in computing power, simulation capabilities, and observing technology.
Combined, this allows us to go farther than before. Instead of simply simulating the overall shape and mass of the galactic halo, we can simulate what both the dark matter and the normal matter distribution should look like for the substructures inside the halo as well. This includes individual galaxies, their halos, gas clouds, satellite galaxies, and even small clumps of dark matter.
These theoretical predictions would also yield different observational signatures. Dark matter will form structures on different scales — substructures of different masses, sizes, and numbers within a large halo — dependent on its mass, temperature, and any potential self-interactions it may have. In January of 2020, a study came out constraining these properties of dark matter based on a sample of strong gravitational lenses that all produced quadruple images.
However, the most massive systems don’t generally have those serendipitous configurations. Instead, we have to rely on mass reconstructions based on more general features produced by these gravitational lenses: arcs, rings, galaxy shape distortion, etc. The simulations will predict, based on what we think we know about dark matter, what types of distortions should be present (and at what level), while the observations allow us to directly infer what the physical dark matter distribution is.
The picture you should have in your head is like this:
- the large dark matter halo that surrounds the galaxy acts like one giant lens,
- with the individual galaxies inside each having their own halo, acting like smaller lenses embedded in the large one,
- with the dark matter substructure within each galaxy and as part of the cluster itself playing an additional role, creating a large number of small-magnitude lenses as well.
Theoretically, dark matter is most often modeled as completely cold, collisionless, and with no interactions other than gravitational interactions. Most of the simulations that have been coded are based under those assumptions, with the largest uncertainties arising from the structures on the smallest scales. But over recent years, observations have caught up to these predictions, allowing us to compare theory (in the form of numerical simulations) and observations at last.
In a new study that was just published earlier this month, observational cosmologists report their results from studying 11 massive galaxy clusters with both ground-based and space-based observatories, where they were able to reconstruct models for the magnitude and number of the various lenses responsible for the signals they saw. On large scales, the simulations and observations lined up very well. But in order to reproduce the details of the observed lensing signatures, the dark matter substructures need to be much richer than simulations predict.
The results are neatly summarized by the study’s authors as follows:
“We report that observed cluster substructures are more efficient lenses than predicted by [cold dark matter] simulations, by more than an order of magnitude.”
Somehow, for some reason, we see a much greater amount of lensing effects arising on very small scales than simulations predict. Either something that we don’t understand is biasing our simulations on small scales, or — just possibly — dark matter is doing something more interesting than just being cold and collisionless.
In many ways, this is the greatest possible type of clue that cosmologists seeking to understand the nature of dark matter could hope for. Simulations have been yielding predictions that don’t quite match up with the details we observe, particularly on very small (sub-galactic) cosmic scales, for approximately 25 years. While adding one simple ingredient — cold, collisionless, invisible dark matter — can simultaneously explain a wide variety of cosmic observations, they’ve often left us wanting more on these small cosmic scales.
Perhaps this is the clue we need. If dark matter has any additional type of interaction in its nature, astrophysical observations like these new cluster measurements could point us in the right direction to uncover exactly what it is. Without the ability to directly detect whatever particles are responsible for dark matter, this interplay of numerical simulations and observed data might be our best path towards solving this mystery. Based on this novel lensing data from rich, massive galaxy clusters, we might at last be one step closer to understanding the true nature and properties of dark matter.
Starts With A Bang is written by Ethan Siegel, Ph.D., author of Beyond The Galaxy, and Treknology: The Science of Star Trek from Tricorders to Warp Drive.