Was Dark Matter Really Created Before The Big Bang?
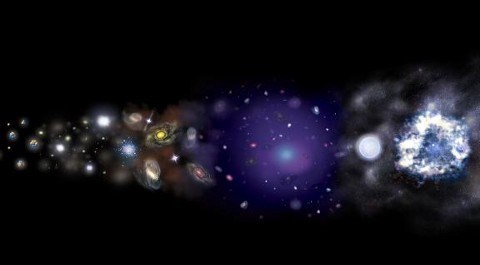
Maybe, but that probably doesn’t mean what you think it does.
Of all the unsolved puzzles in the Universe, perhaps the most confounding is the dark matter problem. If we look at the matter we have in our Solar System, even in the highest-energy and highest-precision labs on Earth, everything we’ve ever observed requires nothing more than the particles of the Standard Model and the bound structures (protons, atoms, molecules, etc.) that they give rise to. Our local corner of the Universe requires nothing more.
But on larger scales — like that of a galaxy, a cluster of galaxies, or the entire cosmic web — normal matter can no longer explain what we see on its own. Whether we’re looking at a spiral galaxy rotate, individual galaxies moving in a massive cluster, or simulating how the large-scale structure of the Universe forms, we cannot get the right answer without adding an enormous amount of extra mass: 5 times as much as the normal matter we infer. That mass must not absorb or emit any light, and hence it’s known as dark matter. But what is dark matter, and when did it arise in our Universe? That’s the big question we’re trying to answer.
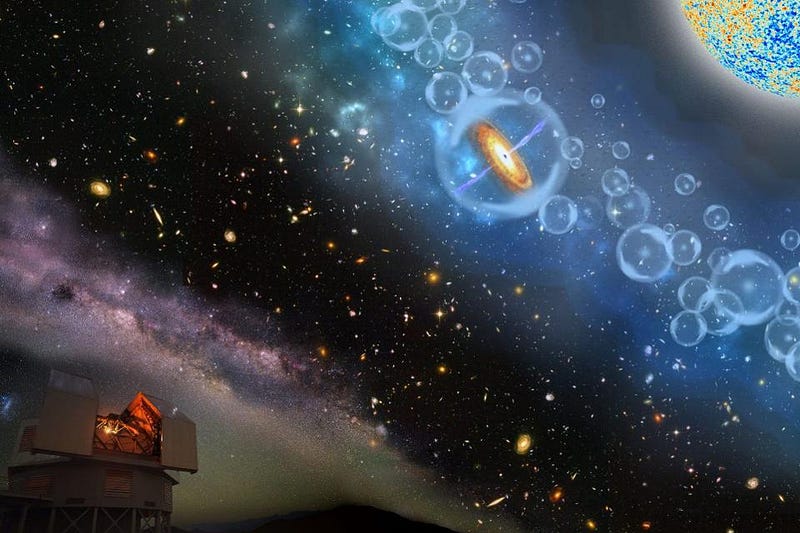
Astronomers have developed an inordinate number of methods to probe the Universe, and all of them point towards one consistent picture of our cosmos. Over the past 13.8 billion years — the amount of time that’s passed since the start of the hot Big Bang — our Universe has expanded, cooled, and gravitated from its initially dense, hot, and almost-perfectly uniform origin.
Today, our observable Universe is enormous in scope: some 92 billion light-years across. It’s filled with trillions of galaxies, clustered together in a great cosmic web, bathed in the Big Bang’s leftover radiation at a minuscule temperature of just 2.73 K. But the biggest surprise is that the particles and fields we know of are insufficient, on their own, to explain the Universe we see. All the matter and radiation we know of, even combined, adds up to just 5% of the energy in the Universe. Two mysterious entities, dark matter (27%) and dark energy (68%), compose the rest.
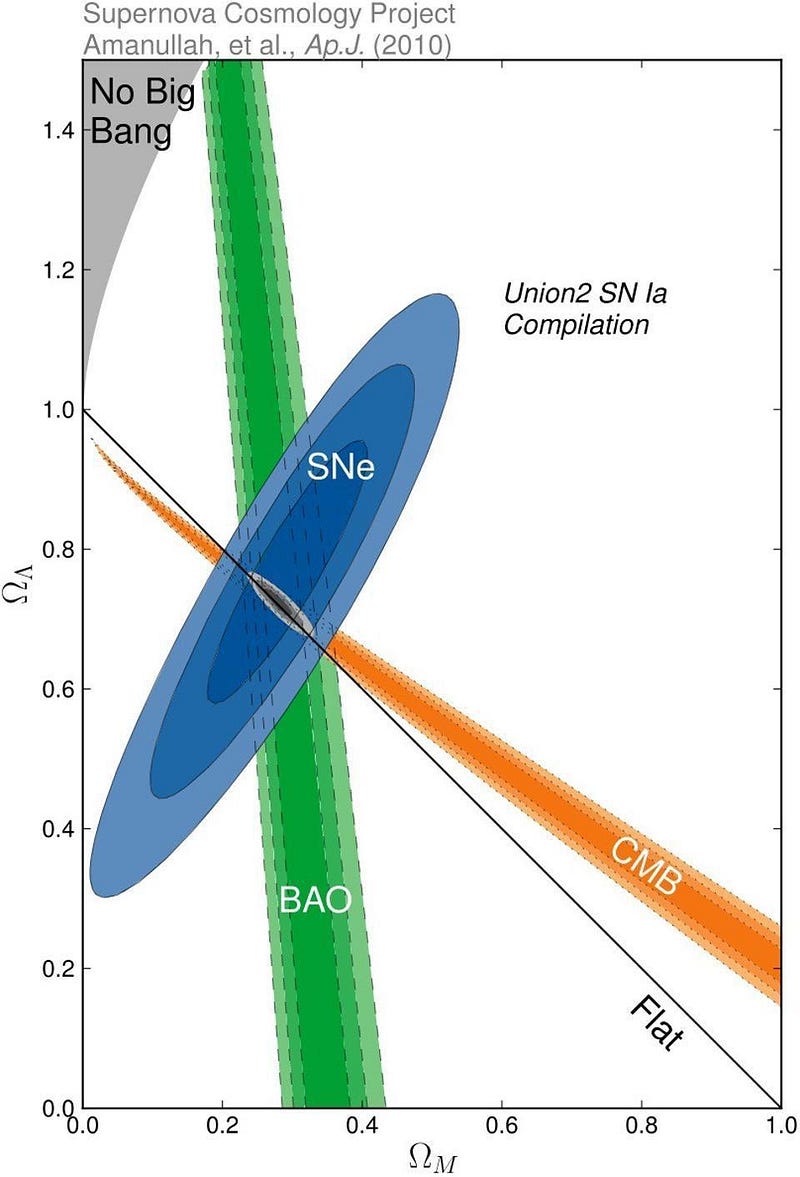
Understanding what those dark components of the Universe are, including where they come from, are some of the great unsolved problems of the 21st century. There are a few things we can infer about both dark matter and dark energy from the observations we’ve accumulated.
- Dark matter: it must have begun uniformly distributed throughout the Universe, with the same initial spectrum of density fluctuations that normal matter possessed. It must have been born cold (i.e., it was moving slowly compared to the speed of light even at early times), and it must not collide or interact (above a certain constrained threshold) with itself or any of the Standard Model particles. And it’s responsible for most of the clumping and clustering in the Universe: its gravitational effects are five times as important as the effects if normal matter.
- Dark energy: we know very little about this. It appears to be completely uniform and not to clump or cluster at all, and it appears to be 100% consistent with a cosmological constant, or of being a form of energy inherent to the vacuum of space itself. Its big effect is twofold, causing the Universe to be spatially flat and driving the accelerated expansion of the Universe, two facts that cannot be explained without it.
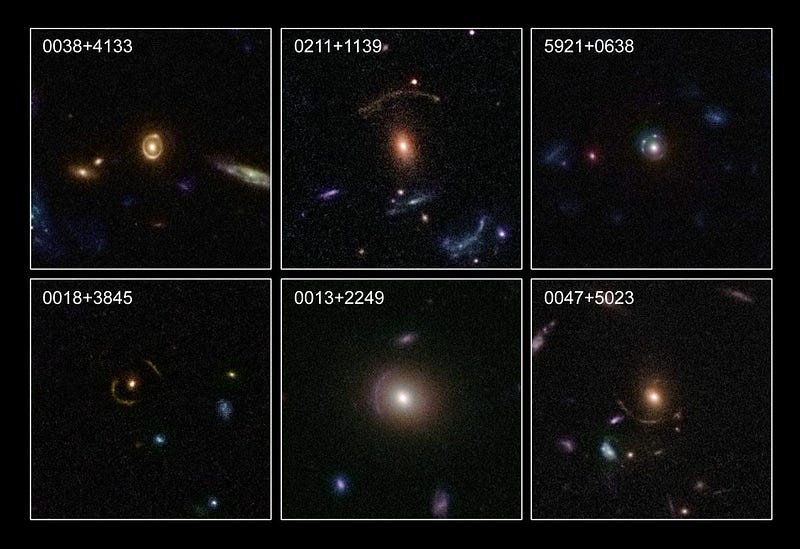
The evidence that exists for dark matter is overwhelming and comes in an enormous suite, but suffers from a distinct drawback from the ideal scenario: it’s all indirect. We can observe the effects that dark matter has on the radiation and the normal matter in the Universe, and come up with many independent measurements that all point to that same 5-to-1 picture of the dark matter to normal matter ratio. In particular:
- the fluctuations in the cosmic microwave background,
- the way galaxies cluster together on the largest scales,
- gravitational lensing of isolated, X-ray emitting galaxy clusters,
- Big Bang Nucleosynthesis and the early-time abundance of the light elements,
- measurements of colliding galaxy groups and clusters,
- peculiar velocities of interacting galaxy pairs,
and many other sets of observations all necessitate the existence of dark matter. Even if we’d never measured an individual galaxy’s rotation — which also supports the existence of dark matter — there’s no way to explain all of what exists without dark matter.
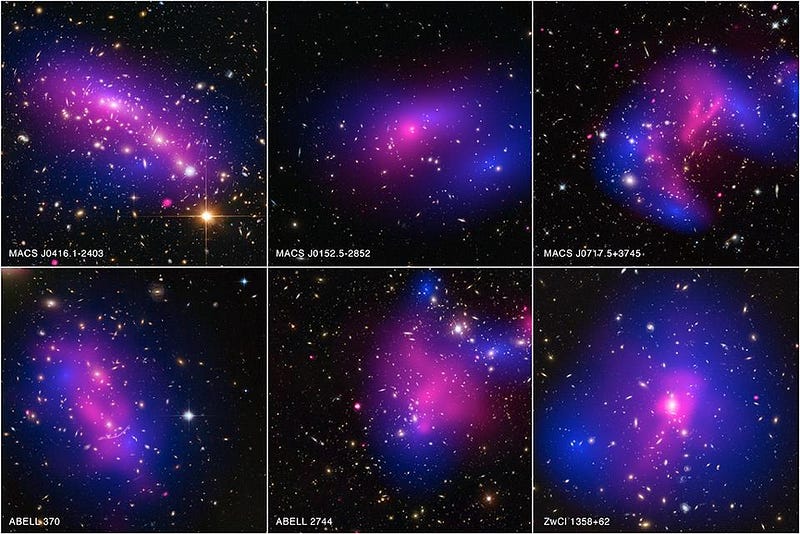
There have been many attempts to directly detect whatever particle might be responsible for dark matter, but all the searches that have ever been carried out have either come up empty or have yielded results where the signal is only dubiously attributed to dark matter. Building large, underground detectors that look for exotic recoils arising from massive particles have placed very stringent constraints on dark matter’s cross-section in a specific mass range, but have never seen such a particle.
Similarly, axion detectors (which search for very light dark matter) have never seen an axion; new “dark sector” particles failed to appear at the LHC; even massive neutrino detectors haven’t seen a signal that couldn’t be explained by neutrinos combined with the known and expected backgrounds. Dark matter is a necessary ingredient of our Universe, but this failure to directly detect it means we don’t know its particle properties, assuming it even is made up of particles.
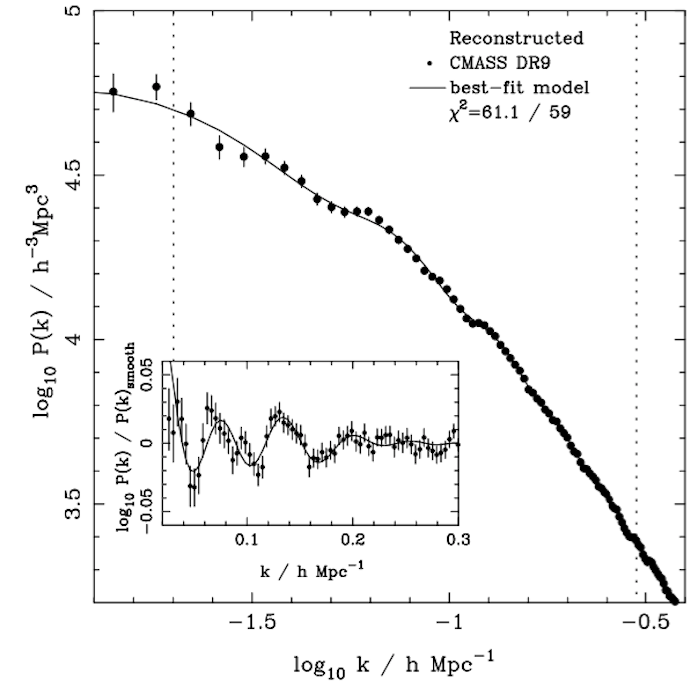
So if that’s what the observational data points towards, what can we say about where dark matter comes from? A recent headline that made quite a splash claimed that dark matter may have originated before the Big Bang, and many people were confused by this assertion.
It might seem counterintuitive, because the way most people conceive of the Big Bang is as a singular point of infinite density. If you say the Universe is expanding and cooling today, then you can extrapolate it back to a state where all the matter and energy was compressed into a single point in space: a singularity. This corresponds to an initial start time for our Universe — the beginning of our Universe — and that’s the Big Bang.
So how could something that exists in our Universe, like dark matter, have originated before the Big Bang? Because the Big Bang wasn’t actually the beginning of space and time.
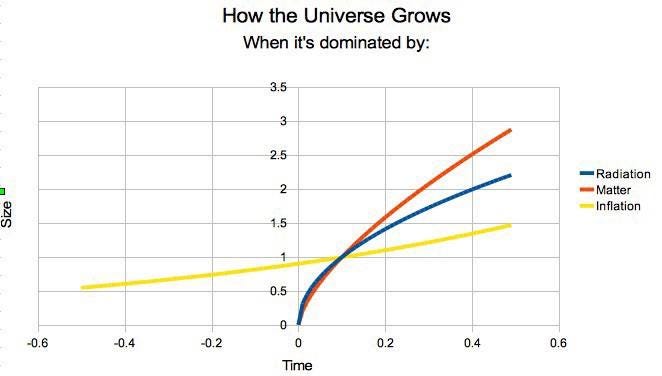
In fact, we’re not even sure whether space and time had a beginning or not, because that extrapolation back to a singularity is now known to conflict with observations. Instead, if we were to extrapolate backwards in time, we’d find that the Universe becomes hotter, denser, and more uniform, but only to a point. Because of the detailed observations we’ve made, especially of the cosmic microwave background (the leftover glow from the Big Bang), we can affirm that there is a maximum temperature that the Universe reached during the hot Big Bang, and that temperature is orders of magnitude below the Planck scale.
In other words, there must have been a different state that preceded and set up the Big Bang. That’s the role cosmic inflation plays and the gap it fills in: inflation is something that occurred in our Universe prior to the hot Big Bang, setting it up and giving the Universe many properties we now observe it to possess.
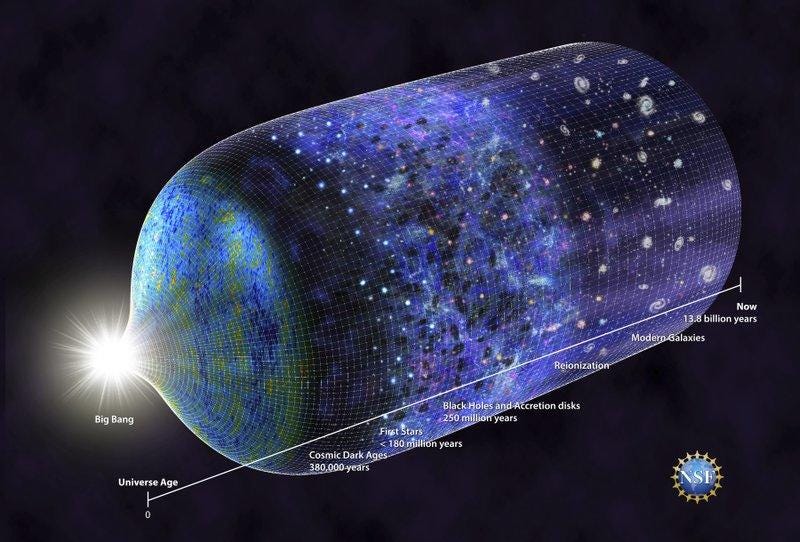
If all of this is true — and it’s the best working model of the Universe that modern science has — then when was all the dark matter in the Universe created? This is where things get interesting, because there are only a few general options, and they all come with caveats. Here are the best options.
- During inflation, before the start of the hot Big Bang.
- During reheating: the transition between inflation and the hot Big Bang.
- During the earliest, most energetic stages of the hot Big Bang.
- During a later phase of the hot Big Bang, due to a phase transition.
That’s it; these are the only options, and they all have drawbacks.
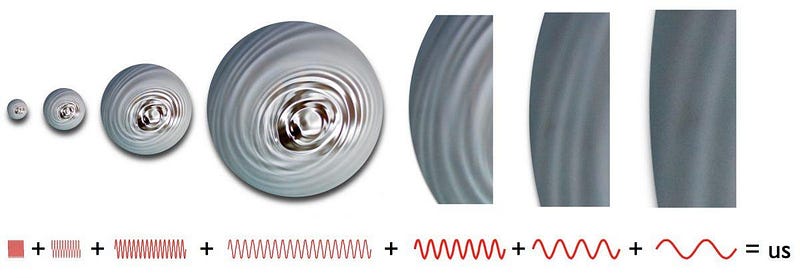
Any particles or field excitations produced during inflation are at risk of being inflated away, as the exponentially expanding nature of an inflationary spacetime can take any two particles arbitrarily close together and inflate them away to be separated by hundreds of billions of light-years on timescales of ~10^-33 seconds. You need to devise a way to retain those relics, which is an extra burden on your theory; the new paper claiming to create dark matter before the Big Bang models this as a new fundamental scalar field in the Universe.
You could try to create dark matter during reheating: the end of inflation where that field energy gets converted into particles: matter, antimatter and radiation. You’d have to create a coupling between the inflationary field and whatever new dark matter field you postulate, which is easy to write down but difficult to extract predictions for.
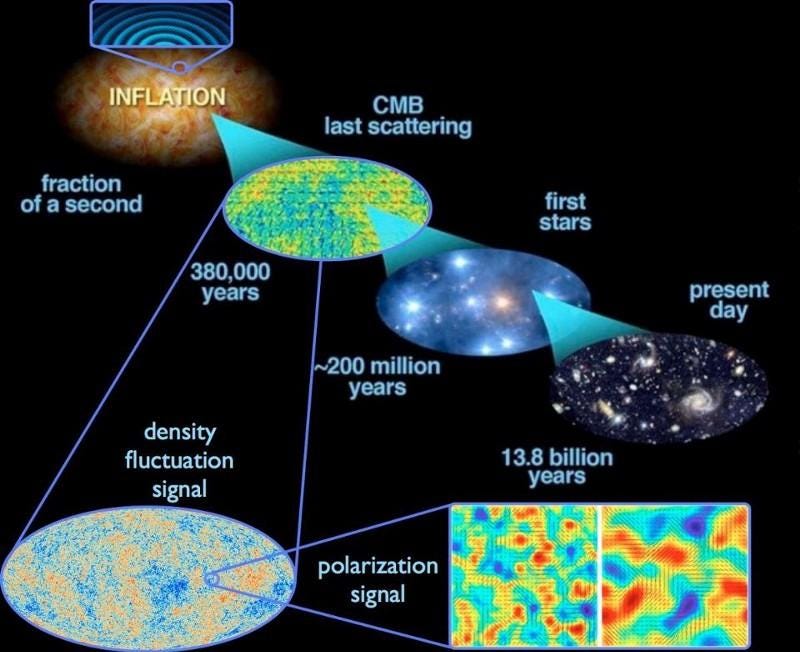
But most of the models of dark matter involve hypothesizing a mechanism of particle creation that occurs after the Big Bang. These models lend themselves to testability much more easily, as they predict particles with finite masses, interaction cross-sections, and directly detectable signatures. Other models offer only indirect signatures, but a thermal relic particle (like a WIMP) or a particle pulled out of the vacuum and given mass by a phase transition (like an axion) offer mechanisms for direct detection as well.
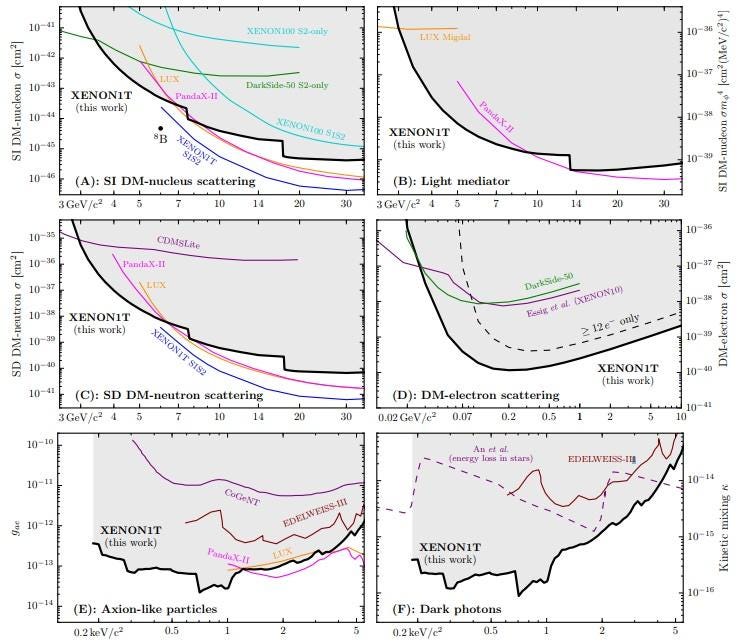
Even though we don’t know exactly what dark matter is, we have a lot of evidence that it exists, and we can do a remarkable job of inferring many of its properties and placing limits on many others. But until we actually know what dark matter is, we have to keep our minds open to all the possibilities, and look for whatever useful scientific signals might be imprinted in our Universe.
Dark matter could have come before or after the Big Bang, but not before the beginning of time and space. When splitting hairs between the options for where dark matter arose in our distant past, a fraction of a second can make all the difference in the cosmos.
Ethan Siegel is the author of Beyond the Galaxy and Treknology. You can pre-order his third book, currently in development: the Encyclopaedia Cosmologica.