Throwback Thursday: Finding the Universe’s first atoms
How we discovered what the Universe was made of when it first formed.
Image credit: X-ray: NASA/CXC/PSU/K. Getman et al.; IRL NASA/JPL-Caltech/CfA/J. Wang et al.
“The nitrogen in our DNA, the calcium in our teeth, the iron in our blood, the carbon in our apple pies were made in the interiors of collapsing stars. We are made of starstuff.” –Carl Sagan
When we look out into the distant Universe, we’re also looking back into the Universe’s past. The farther away an object is, the longer it’s taken its light to travel from it to our eyes. And each time we observe something farther away than anything we’ve seen before, we’re looking farther back into the past — closer to the Big Bang — than ever before.
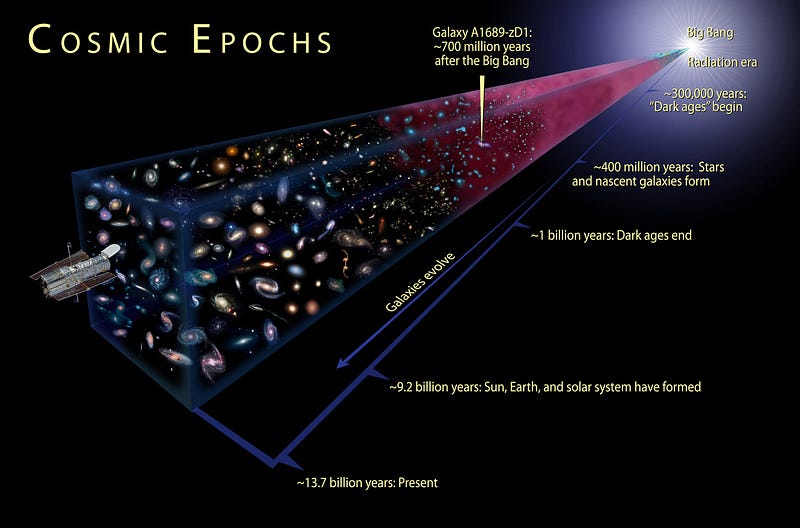
As telescopes get larger and more sensitive, and as exposure times get progressively longer, we’re able to uncover fainter, more distant objects in the Universe. But even in theory, there’s a limit.
The earliest thing we’ll ever been able to see — as far as light goes — is the Cosmic Microwave Background, or the leftover glow of radiation from the Big Bang. When we observe this radiation background, which was emitted when the Universe finally cooled to low enough temperatures that neutral atoms could form, we’re getting a snapshot of the Universe as it was from when it was only 380,000 years old!
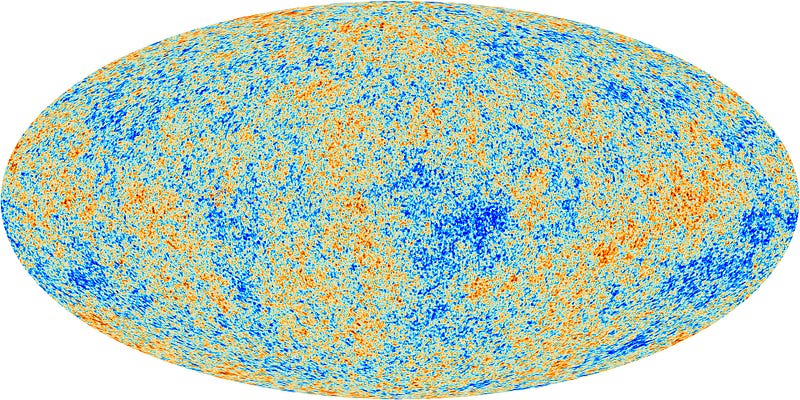
The reason this is the limit to what we can see is because prior to this epoch, the Universe was ionized, and was a sea of unbound electrons, protons, and a few other light nuclei. The electrons are what’s important for our purposes: photons can’t travel very far without running into an electron, which absorbs it and re-emits it, thanks to the phenomenon of Compton/Thomson scattering.
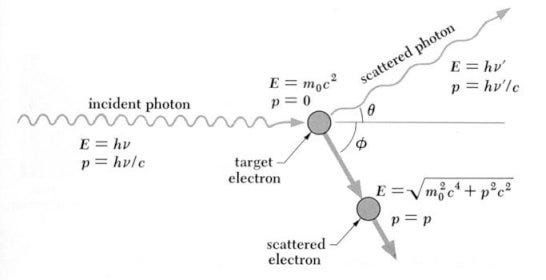
So we can’t directly see what came before the Universe was 380,000 years old, but there was plenty of interesting physics happening before then that we’d love to be able to test! You see, there is a theoretical prediction of the Big Bang that comes from even earlier times; it is perhaps the earliest testable prediction we have about the Universe!
The Big Bang not only tells us when we should form atoms for the first time, it tells us what types of atoms we expect there to be.
How so? Let’s take you back to the earliest times we can speak of where we still have near-100% confidence in our physics.
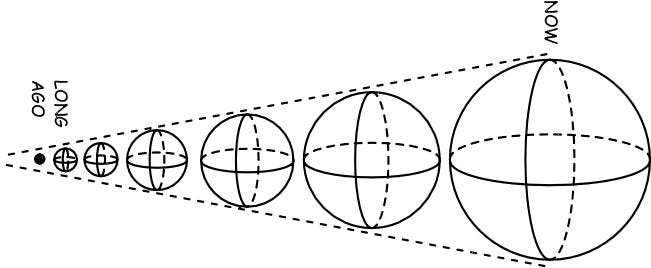
Remember that the Universe is expanding and cooling even now, which tells us that it was hotter and denser in the distant past! Sure, when the Universe was less than 380,000 years old, it was too hot to have neutral atoms, but what if we go to even earlier times?
At some point it was too hot and dense to even have nuclei, and at some even earlier point than that, the Universe was too energetic to even have individual protons and neutrons! Back when the Universe was a tiny fraction of a second old, all we had was a sea of quarks, gluons, leptons, antileptons and ultra-hot radiation, swirling around in the primordial soup of the Early Universe!
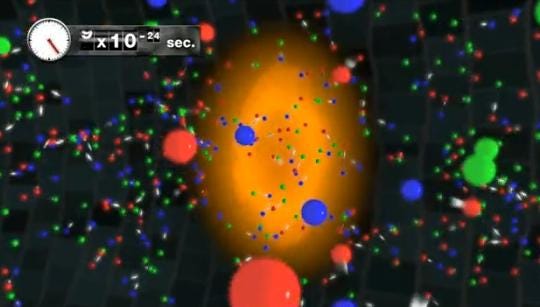
In this state, everything collides extremely rapidly, and is in a state of thermal equilibrium, where all the particles near one another wind up with the total kinetic energy distributed among them in an equilibrium configuration. Under these conditions, creation and annihilation of particle-antiparticle pairs happens rapidly.

However, nearly all of the particles in existence here are unstable! As the Universe expands and cools, the heavy leptons and quarks decay away, the excess matter and antimatter find one another and annihilate, and the leftover quarks (up-and-down, in roughly equal amounts) cool down enough to condense into individual protons and neutrons. By time the Universe is about 10 microseconds old, protons and neutrons exist in roughly equal numbers.
However, the Universe is also filled with electrons and anti-electrons, better known as positrons. Every time a proton collides with an energetic-enough electron it produces a neutron (and a neutrino), while every time a neutron collides with an energetic-enough positron, it produces a proton (and an anti-neutrino). Initially, these reactions proceed at about the same speed, giving a Universe whose normal matter is made up of 50% protons and 50% neutrons.
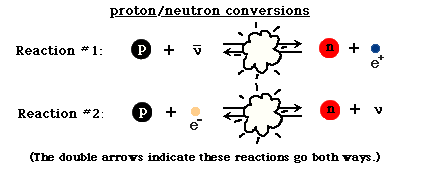
But due to the fact that protons are lighter than neutrons, it becomes more energetically favorable to have more protons than neutrons in the Universe. (See here for some quantitative notes.) By time the Universe is three seconds old and the interconversions have mostly stopped, the Universe is more like 85% protons and 15% neutrons. And at this time, it’s still hot and dense enough that the protons and neutrons attempt to undergo nuclear fusion, into deuterium, the first heavy isotope of hydrogen!
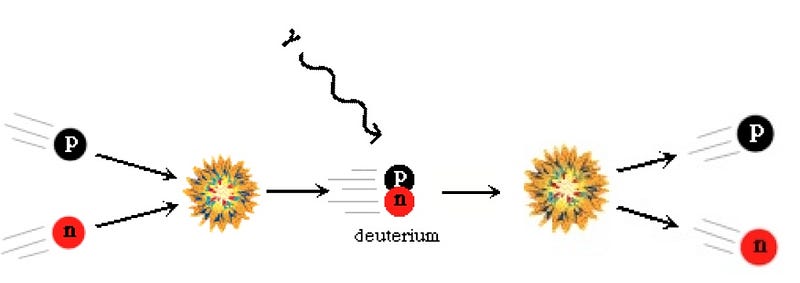
But the Universe is filled with over a billion photons for every proton or neutron in it, and the temperature is still far too high to produce deuterium without it being immediately destroyed. So you wait and you wait, until the Universe to cool down enough to make deuterium withoutimmediately blasting it apart. In the meantime, you’re faced with the unpleasant fact that the neutron is unstable, and some of your neutrons decay away, into protons, electrons, and an antineutrino.
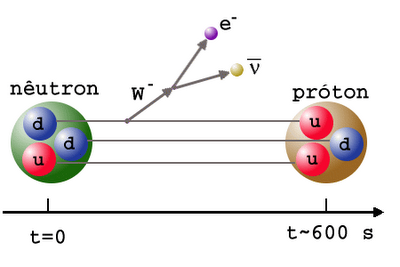
Finally, when the Universe is somewhere between three and four minutes old, the photons have cooled off enough that they can no longer blast deuterium apart faster than the protons and neutrons can meet to form it; the Universe finally passes through the deuterium bottleneck. At this point, thanks to the decays, the Universe is somewhere around 88% protons and only 12% neutrons.
Once you can make deuterium, the Universe wastes no time adding protons and/or neutrons to it in rapid succession, climbing up the elemental ladder to make tritium or Helium-3, and after that, the very stable Helium-4!
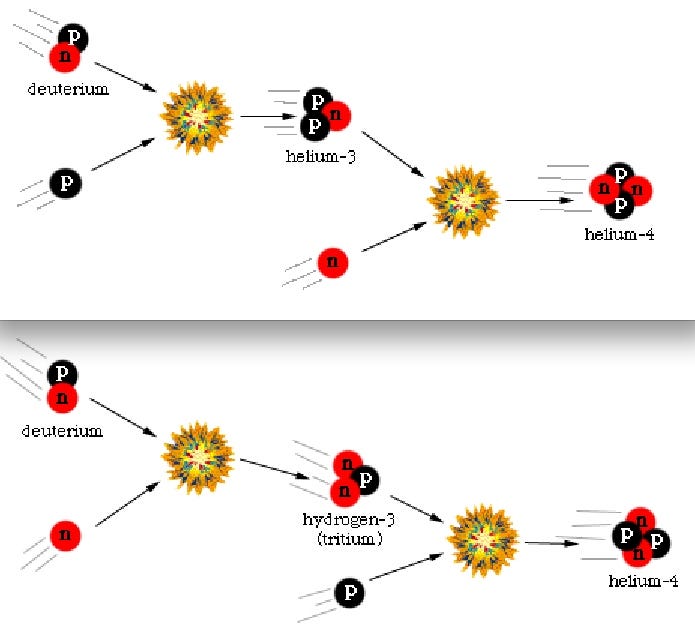
Nearly all of the neutrons wind up in Helium-4 atoms, which winds up as around 24% of the atoms, by mass, after this nucleosynthesis. Hydrogen nuclei — which are just single protons — make up the other 76%. There’s also very small fraction (between 0.001% and 0.01%) in Helium-3, tritium (which decays into Helium-3) and Deuterium, and an even smaller fraction winding up in some form of Lithium or Beryllium, from nucleosynthesis of those rare isotopes with a Helium-4 nucleus.
But because of a combination of factors — the lack of a stable mass-5 or mass-8 nucleus, the coolness / relatively low density of the Universe by this time, and the strong electrical repulsion of the heavier isotopes — nothing heavier forms.
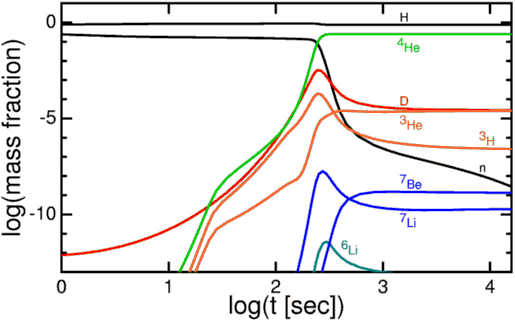
And so these are the elements that are predicted by the Big Bang. With our knowledge from the Cosmic Microwave Background, we can determine — to incredible precision — exactly how much Helium-4, Helium-3, Deuterium, and Lithium-7 should be around today. This prediction — the initial abundance of the light elements — is one of the greatest predictions to come out of the Big Bang model.
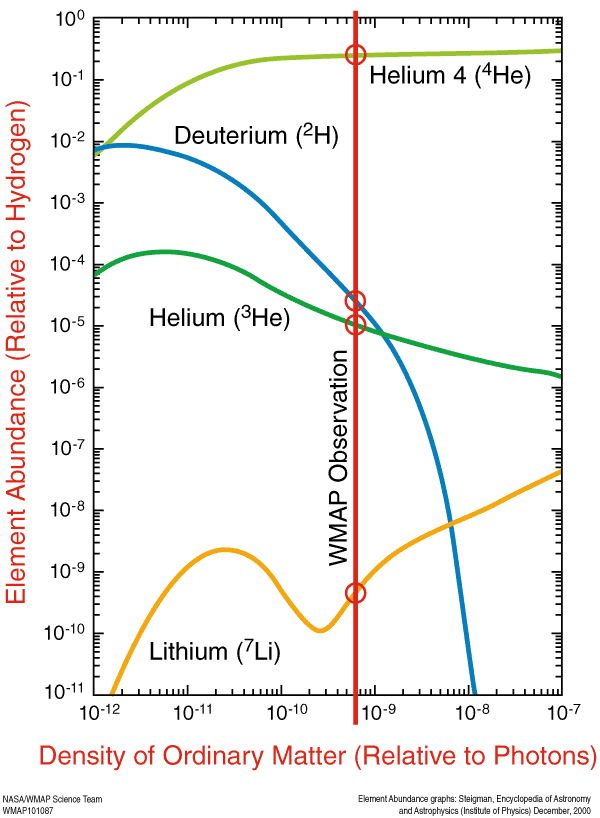
After that, the Universe simply expands and cools, while the unstable isotopes (like tritium) decay into stable ones, until these atomic nuclei — forged in the nuclear furnace of the Big Bang — can safely capture electrons and become neutral atoms.
At least, that’s what the theory says. Of course, seeing these first atoms, and measuring their abundances, is especially challenging, but something we’d really want to do to confirm this picture. Why’s that? Let’s take a look at what you can see if you look out — and back — into the Early Universe.
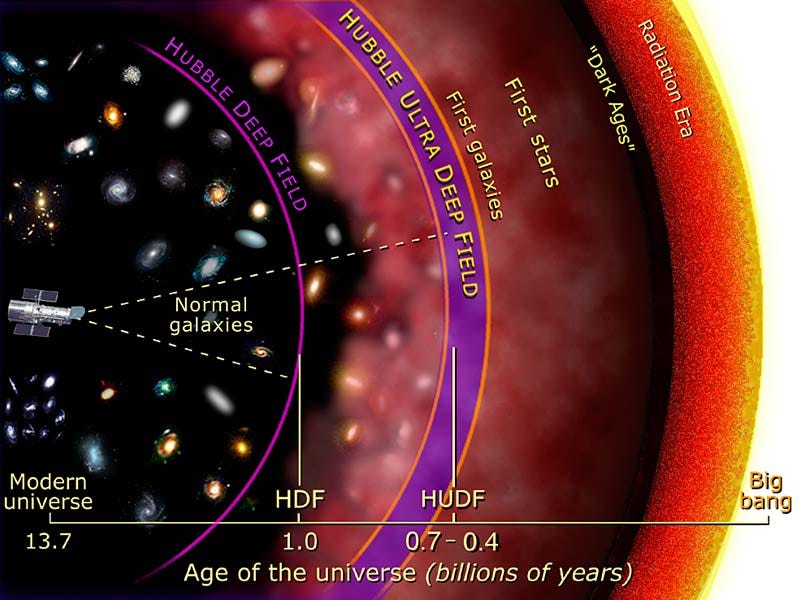
What we want to see are the very first atoms: the ones that exist back in the cosmic dark ages of the Universe. But this presents a tremendous difficulty.
The way we detect elements in the Universe is from their atomic transitions, which either give emission lines if the atoms are hot enough to have their electrons in an excited state drop down to a lower-energy state, or absorption lines if the atoms are in a cold/low-energy state, but there is a hot source behind them whose photons of a particular energy are absorbed.
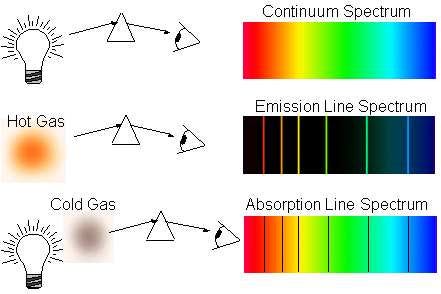
The problem, of course, is that these “dark age” atoms are too cold themselves to emit those emission lines, and the radiation coming from behind them is too low in energy to induce these absorption lines! So again, we have to wait for gravitation to work its magic on these atoms, and to gravitationally attract enough of them into one place so that we can get to work on making something energetic enough to induce these atomic absorption features!
After enough gravitational collapse happens, the Universe becomes dense enough, in spots, to finally form stars for the first time! The regions that become densest the fastest form stars first — as soon at 50–150 million years after the Big Bang — while other regions remain neutral, devoid of stars, and pristine for longer.
The first problem is, when we create these first stars, the neutral atoms block the light from them, just as a thick cloud of interstellar gas can block the starlight from behind it.
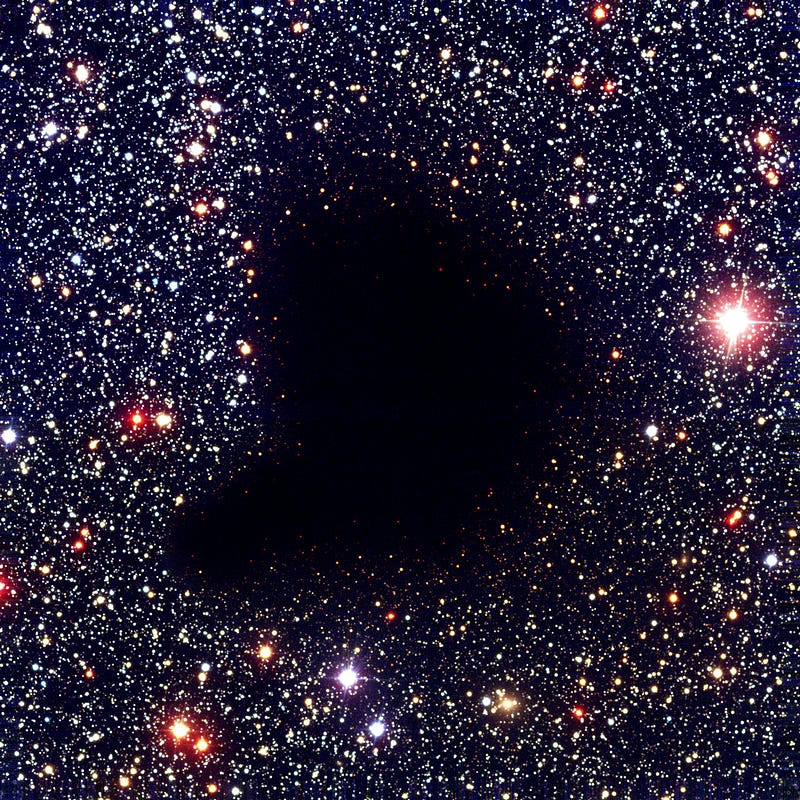
So what we’d need, if we even want to see the light coming from these stars (or any light source) in the first place, is to get rid of these neutral atoms. And the way you do that is to form enough stars throughout the Universe that you — for all intents and purposes — reionize the vast majority (99%+) of the neutral atoms in it.
Thankfully, the Universe does this all on its own, and it does this after less than one billion years.
The other problem is that once gravitational collapse happens and you form the first stars, these stars — in very short order — not only pollute the Universe around them with the heavier elements they create, they also destroy these tenuous light elements — deuterium, lithium, and helium-3 — that we’d like to measure!
Sounds like a catch-22, doesn’t it? How can we measure these first, pristine atoms if we can only measure atoms at all after a billion years of stuff has happened to pollute the atoms in the Universe?!
As it turns out, there is one hope.
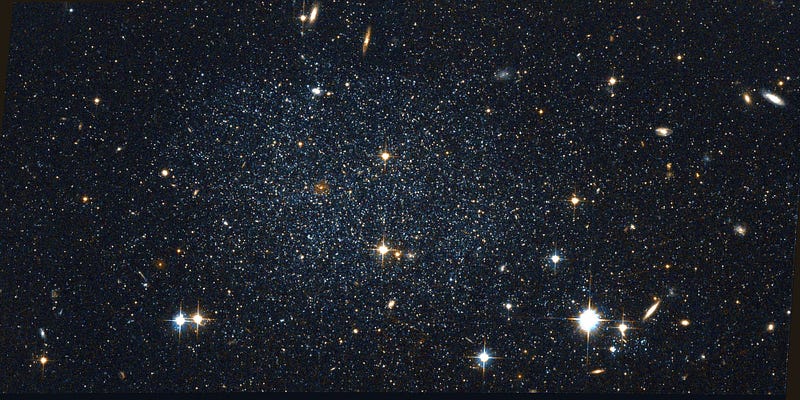
The Universe has — although they’re very difficult to find — ultra-low-mass, isolated galaxies, like the Antlia Dwarf Galaxy, above.
In theory, extraordinarily isolated clumps of matter, whose mass totals something like only 0.0001% of our Milky Way Galaxy, may survive without forming any stars at all, and without being polluted by any nearby post-stellar mass, for well over a billion years. But if we wanted to find one, we’d have to be incredibly lucky. From the time the Big Bang first was proposed as a theory in the 1940s, we didn’t have that luck for years, and then decades, and then for generations.
But then 2011 came along, and we’ve had two strokes of luck that serendipitously have given us the luck we’ve been waiting for!
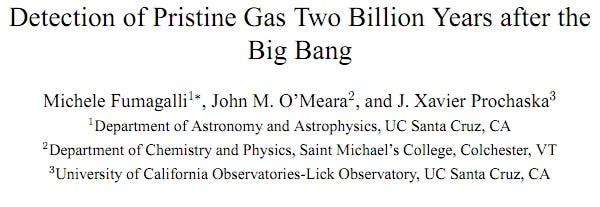
The brightest, most luminous objects visible in the farthest reaches of the distant Universe are quasars, a good number of which are visible right at the very end stages of reionization — when light becomes transparent to matter — in the Universe. In a serendipitous stroke of good luck, after 58 years of quasar spectroscopy, the above team of Fumagalli, O’Meara and Prochaska found two clouds of pristine, unpolluted gas from the Big Bang in the spectra of their quasars!
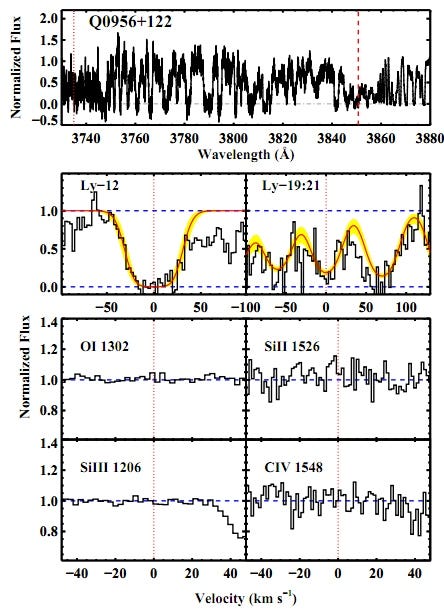
The top part of the above image, from the Fumagalli et al. paper, is the actual quasar spectrum. That zigzag pattern, everywhere you see a downward dip, is the signature of an absorption line! In this particular case, the absorption lines show a pattern characteristic of a cloud of neutral hydrogen gas at a redshift just slightly greater than 3, or about a time of 2 billion years after the Big Bang. (And about 1 billion years after the initial light left this quasar!)
However, the accompanying “pollutant” elements normally found as evidence of prior stars — Carbon, Oxygen, Silicon, etc. — are all not only absent, they’re absent to the extent that we can quantify that there’s less than 0.01% of the amount found in our Sun. (And that’s an upper limit.) Keep in mind, the next most-pristine gas cloud we’ve ever found in the Universe has at least 0.1% of the heavy elements found in the Sun; that’s a lower limit. So we’re talking something that’s more than 10 times purer than anything else we’ve ever found!
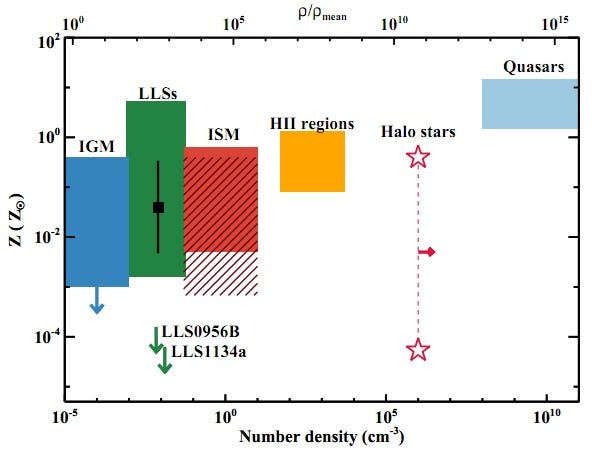
So this is not only the least polluted, most pristine sample of atoms we’ve ever found, it’s also the newest, best test we’ve ever conducted as to whether the abundances of these light elements — from the strength of their spectral absorption lines — matches up with the predictions of the Big Bang!
The results? Have a look at the most pristine, left-most point on the graph below (and keep in mind that these are 68% confidence-level error bars); it’s the most trustworthy data ever taken on this topic!
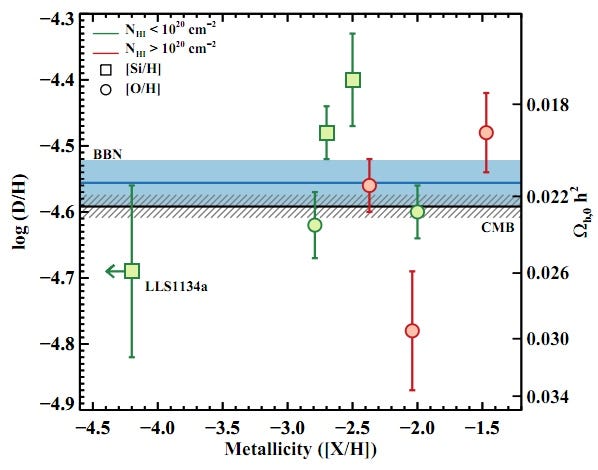
As the paper itself states:
For quasar sight lines, the measured log(D/H) = −4.55 ± 0.03 translates into Ω_b,0 h^2 (BBN) = 0.0213 ± 0.0010, which is fully consistent with the value inferred from the Cosmic Microwave Background (CMB) power spectrum Ω_b,0 h^2 (CMB) = 0.02249 ± 0.00057. This excellent agreement between two essentially independent experiments stands as a marked triumph of the Big Bang theory.
The best part? If we want to measure the elements found in these gas clouds better, all we have to do is observe them for longer amounts of time! Yes, we might get lucky again and find even more of these pristine gas clouds (the rule-of-thumb is: if there’s one, it might be a fluke, but if there are two, there are probably many), but even if we don’t all we have to do is look more and more precisely at these quasars, and we can untangle the abundances of the elements found here to ever greater precision!
And that’s how we found the very first atoms in the Universe, and how they — yet again — proved another prediction of the Big Bang correct!
Leave your comments at the Starts With A Bang forum on Scienceblogs!