Eight New Quadruple Lenses Aren’t Just Gorgeous, They Reveal Dark Matter’s Temperature
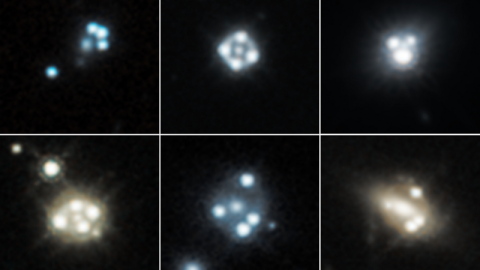
The images themselves will take your breath away, but the science we can extract from them is truly revolutionary and spectacular.
Dark matter may be one of the most mysterious components of our Universe, having eluded direct detection since it was first proposed in the 1930s. Although the astrophysical evidence for its existence is overwhelming — from spinning galaxies, galactic motions in clusters, large-scale structure formation, colliding galaxy groups, the cosmic microwave background and more — we don’t know what its true nature is.
One of the best methods for studying dark matter is through its gravitational effects, particularly in extreme environments: where Einstein’s General Relativity makes unique predictions that differ from Newtonian gravity. Strong gravitational lensing, where intervening masses between us and a distant source creates distorted, magnified, and multiple images of the target, is one of the best probes of matter in general. With a new set of eight strongly lensed, quadruple-image systems, scientists are learning about dark matter’s properties as never before.
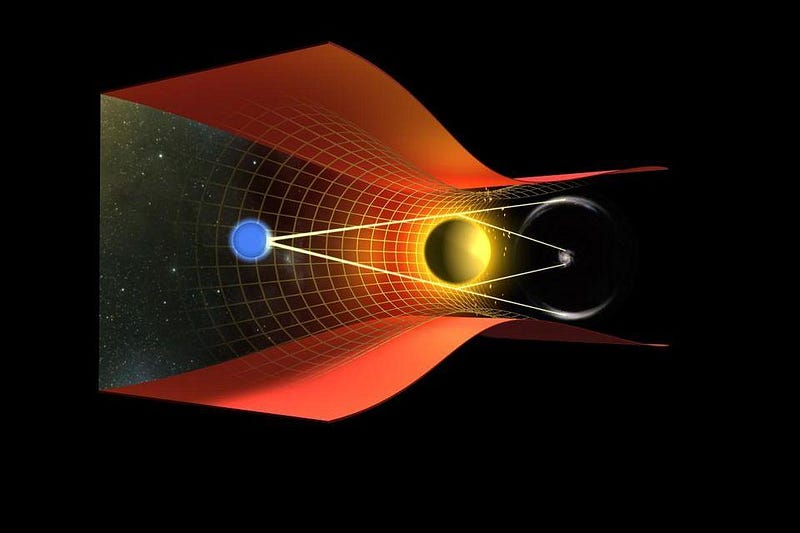
In Einstein’s General Relativity, unlike in Newton’s old theory of gravitation, it isn’t an invisible attraction between masses that causes what we perceive as gravity, but rather the relationship between matter-and-energy and space-and-time. The presence of matter and energy curves the fabric of space, and that curved space affects everything else in the Universe, including light that passes through that very space.
Whenever you have space that’s curved by a large enough amount, it will affect the light traveling through that region in a fascinating array of ways. Instead of flat space, where light must always travel in a straight path between two points, the presence of curved space means that multiple paths can be taken to connect two points in space. If the alignment is absolutely perfect, you can even see the background light get stretched into a circular structure: an Einstein Ring.
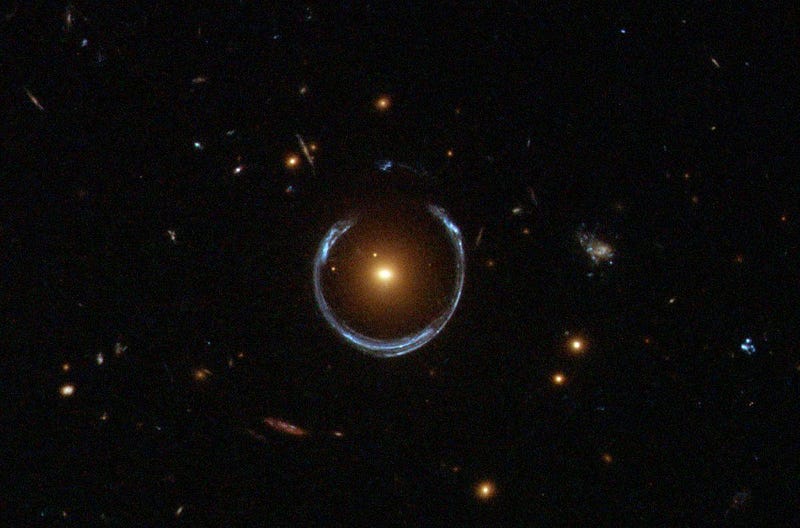
Of course, most of the time the alignment isn’t perfect, and there’s a good reason perfect alignments are rare: the Universe itself isn’t perfect. Which is to say, it’s full of imperfections, governed by the growth of gravitational overdensities that lead to the cosmic web we see today.
We might think about the Universe as being made of galaxies that are grouped and clustered together into filaments that connect at various nexus points, but that would be a mistake. Yes, that’s what our Universe appears to look like to our eyes and instruments, but that’s only the normal matter: the stuff made of protons, neutrons, and electrons. What’s unseen by those techniques is the dark matter, which is 5/6ths of the mass of the Universe, but only forms the diffuse “skeleton” traced out by the cosmic structure that we can observe.
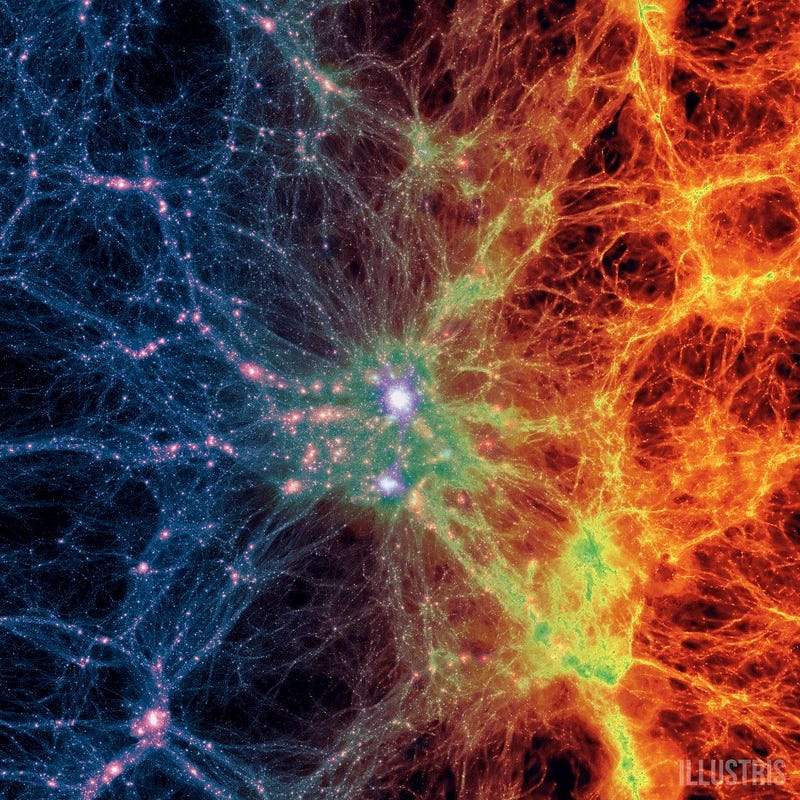
If we go down to very detailed scales, the dark matter situation is even more interesting. Wherever you have dark matter, it doesn’t just make this large, diffuse, fluffy halo on cosmic, super-galactic scales. In addition to that, there are also miniature sub-halos of all different sizes, occurring:
- along the filaments,
- in the locations where galaxies and clusters form,
- between the locations where galaxies exist,
- and superimposed atop all the larger structures — both normal and dark — that exist.
If we were to look at a typical dark matter simulation of a galaxy’s halo, and we superimposed the normal, luminous matter atop it, what we would see isn’t just one enormous dark matter “fluffball,” but a series of smaller-scale dark matter substructure that flows through the galaxy.
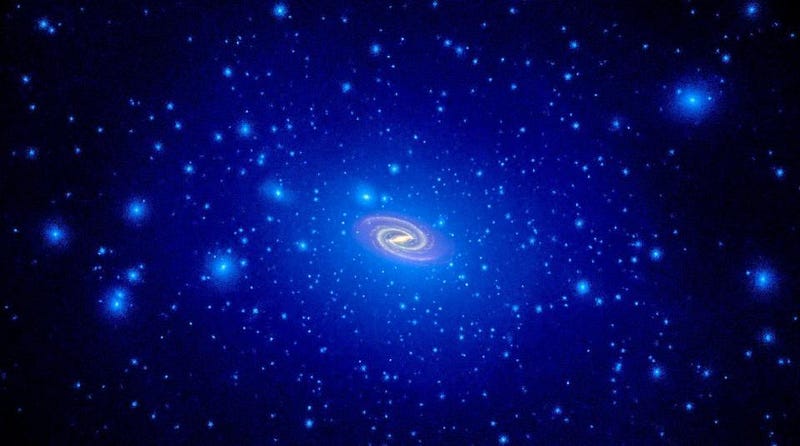
The reason this is important is because the gravitational lensing we observe when we look at strong lensing systems isn’t caused by just one large, smooth source of mass. Instead, the amount and type of lensing signal that we’ll observe is the sum of all the different forms of matter-and-energy that exist along the line-of-sight to a particular object.
One of the most spectacular configurations of a lensing system is where you get a “cross” configuration: four images offset by approximately (but not quite) 90 degrees from one another. Long before the first Einstein Ring was ever found, an Einstein cross showed up, resulting mostly from the gravitational influence of a large non-spherical mass primarily responsible for the strong lensing of a slightly off-center source. The background light gets stretched, magnified, and produces multiple images, a spectacular sight that also allows us to extract some spectacular science.
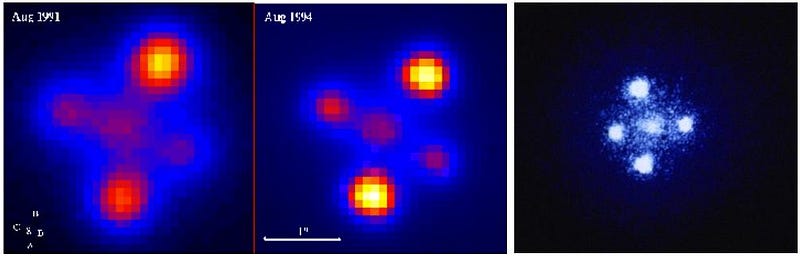
When you take a look at the details of a system configured like this, it doesn’t just depend on the major mass source lensing it, but all of this intricate dark matter substructure arising from these miniature halos as well. By examining exactly how the light from each of the four images is bent relative to one another — something only newly possible with spectroscopic techniques of ionized oxygen and neon signatures — it’s possible to extract information about the types of subhalos that dark matter can form.
Using data from the Hubble Space Telescope, a team including Prof. Anna Nierenberg and PhD candidate Daniel Gilman were able to perform this analysis of large-scale structure, integrated over the line-of-sight, for eight different quadruply lensed systems. By observing the variations due to the substructure, which appears at the level of just a few thousandths-of-a-percent, they were able to gain information about the nature of dark matter.
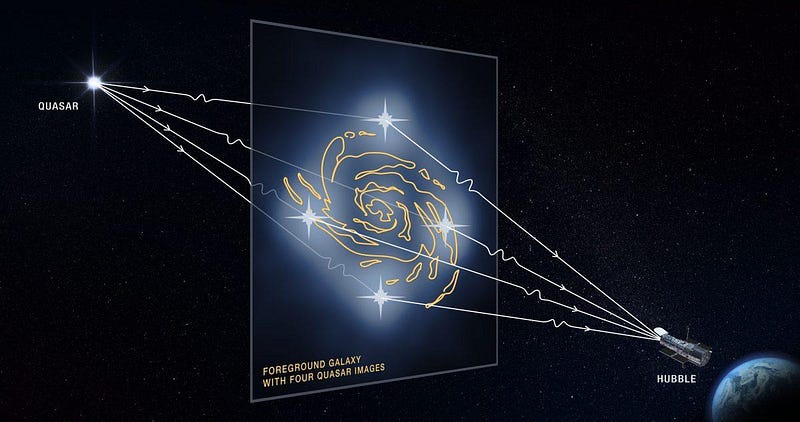
In particular, dark matter could in principle have been born with any amount of kinetic energy and any mass at all. However, in practice, if dark matter were born light and fast-moving, the types of structures that would have formed in the Universe would have been suppressed on the smallest of scales.
When we find evidence for small-scale structures, and we start measuring the properties of those structures, we can begin to place meaningful constraints on how massive and slow-moving dark matter is allowed to be. For example, we know that dark matter cannot be composed of the known neutrinos present in our Universe: that dark matter would be too hot. While we typically talk about cold dark matter, there is still the possibility that dark matter could be warm at some level, possessing significant kinetic energy for whatever mass it has.
Previously, two different methods had been used to place the best constraints on the temperature/mass properties of dark matter, but both required assumptions.
- Tidal streams from the vicinity of the Milky Way provide a probe of substructure and therefore of dark matter’s nature, but these streams rely on assumptions the interplay of normal matter with dark matter, which is highly uncertain in a number of regards.
- The Lyman-alpha forest — where light from distant quasars pass through clouds of gas that partially or wholly absorb the light — enable us to know how small and large structures grow from even very early on in the Universe, but again require assumptions about the gravitational growth of matter and the infall of normal matter into dark matter halos.
The constraints on these are good; if dark matter is a thermal relic (meaning it was once produced with the kinetic energy of the other particles in the early Universe), it must be either more massive than 6 keV or 5.3 keV from these methods, respectfully, assuming all the assumptions are valid. (This is some ~10,000 times more massive than the current bound on neutrino masses.)
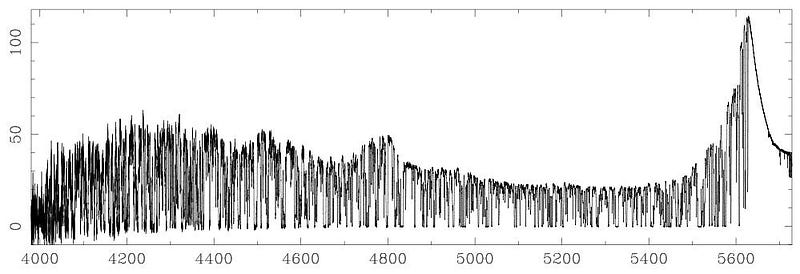
But by leveraging this new method, excellent constraints that are independent of any assumptions about the normal matter in the Universe were obtained. As Daniel Gilman, who presented this research at the American Astronomical Society’s annual meeting stated,
Imagine that each one of these eight galaxies is a giant magnifying glass. Small dark matter clumps act as small cracks on the magnifying glass, altering the brightness and position of the four quasar images compared to what you would expect to see if the glass were smooth.
There was no dependence on the interplay of light and normal matter, or of normal matter with dark matter, instead relying on the curved path that the light must follow alone. From just this work, dark matter, if it’s a thermal relic, must be more massive than 5.2 keV, meaning it could be either cold or lukewarm, but no hotter.
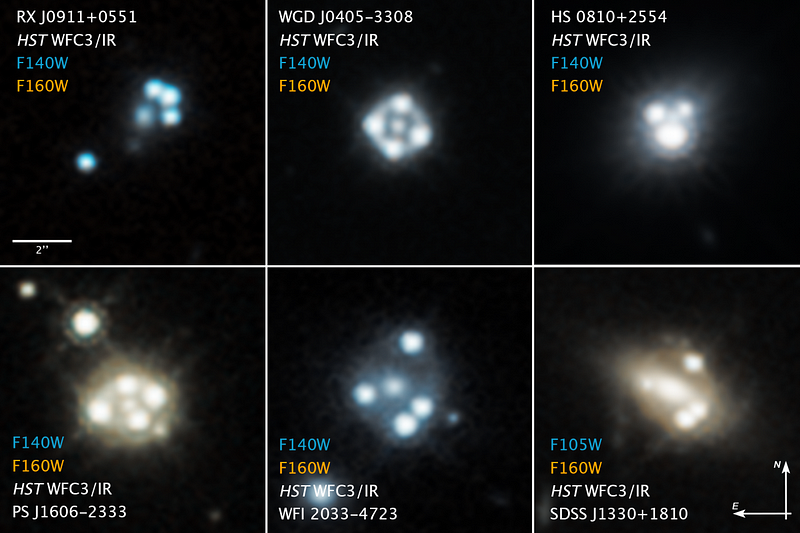
Ever since astronomers first realized that the Universe required the existence of dark matter to explain the cosmos that we see, we’ve sought to understand its nature. While direct detection efforts have still failed to bear fruit, indirect detection through astronomical observations not only reveal the presence of dark matter, but this novel method of using quadruply lensed quasar systems has given us some very strong, meaningful constraints on just how cold dark matter needs to be.
Dark matter that’s too hot or energetic cannot form structures below a certain scale, and the observations of these ultra-distant, quadruple-lens systems show us that dark matter must form clumps on very small scales after all, consistent with them being born as arbitrarily cold as we can imagine. Dark matter’s not hot, nor can it even be very warm. As more of these systems come in and our instruments go beyond what even Hubble’s capabilities are, we might even discover what cosmologists have long suspected: dark matter must not only be cold today, but it must have been born cold.
Ethan Siegel is the author of Beyond the Galaxy and Treknology. You can pre-order his third book, currently in development: the Encyclopaedia Cosmologica.