Ask Ethan: Can A Laser Really Rip Apart Empty Space?
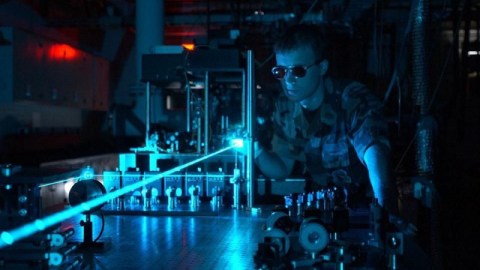
Did you hear the story about how a 100 petawatt laser will finally ‘break the quantum vacuum’? Get the facts.
Empty space, as it turns out, isn’t so empty. The fluctuations in the vacuum of space itself mean that even if you take all the matter and radiation out of a region of space, there’s still a finite amount of energy there, inherent to space itself. If you fire a powerful enough laser at it, can you, as a Science magazine story called it, break the vacuum and rip apart empty space? That’s what our Patreon supporter Malcolm Schongalla wants to know, as he asks:
Science Magazine recently reported that Chinese physicists will start building a 100-petawatt(!!!) laser this year. Can you please explain how they plan to achieve this, and what unique phenomenon this will help physicists explore? Such as, what exactly is “breaking the vacuum?”
The story is real, verified, and a little bit exaggerated in terms of claims that it can break the vacuum, as though such a thing were possible. Let’s dive into the real science to find out what’s really happening.
The very idea of a laser itself is still relatively novel, despite how widespread they are. Originally an acronym standing for Light Amplification by Stimulated Emission of Radiation, lasers are a bit of a misnomer. In truth, nothing is really being amplified. You know that, in normal matter, you have an atomic nucleus and various energy levels for an electron; in molecules, crystals, and other bound structures, the particular separations between an electron’s energy levels dictate which transitions are allow. In a laser, the electrons oscillate between two allowable states, emitting a photon of a very particular energy when they drop from the higher-energy state to the lower one. These oscillations are what produce the light, but for some reason, no one wanted the acronym Light Oscillation by Stimulated Emission of Radiation.
If you can produce either multiple atoms-or-molecules in the same excited state and stimulate their spontaneous jump to the ground state, they’ll emit the same energy photon. These transitions are extremely fast (but not infinitely so), and so there is a theoretical limit to how quickly you can make a single atom-or-molecule hop up to the excited state and spontaneously emit a photon. Normally, some type of gas, molecular compound or crystal is used inside a resonant-or-reflective cavity to create a laser, but you can also make one out of free electrons, semiconductors, optical fibers, and, in theory, even positronium.
The amount of energy that comes out of a laser is limited by the amount you put in, so the only way to achieve extremely high power in your laser is to shorten the timescale of the emitted laser pulse. You might hear the term petawatt, which is 10¹⁵ W, and think this is a tremendous amount of energy. But “petawatts” aren’t energy, but power, which is an energy over a time. A petawatt laser could either be a laser that emits 10¹⁵ J of energy (the amount released by about 200 kilotons of TNT) every second, or could just be a laser that emits one joule of energy (the amount released by burning 60 micrograms of sugar) over femtosecond (10^-15 second) timescales. In terms of energy, these two scenarios are vastly different, even though their power is the same.
The 100 petawatt laser in question hasn’t been built yet, but is rather the next enormous threshold that researchers plan to cross in the 2020s. The hypothesized project is known as the Station of Extreme Light, and is set to be constructed at the Shanghai Superintense Ultrafast Laser Facility in China. An external pump, which is usually light from a different wavelength, excites the electrons in the lasing material, causing the characteristic transition that creates the laser light. The photons then all emege in a tightly packed stream, or a pulse, at a very narrow set of wavelengths. To the surprise of many, the 1 petawatt threshold was crossed way back in 1996; it’s taken nearly two decades to cross the 10 petawatt mark.
The National Ignition Facility in the United States may be what we first think of when we envision high-powered lasers, but this is a bit of a red herring. This array of 192 lasers, focusing on a single point to compress a hydrogen pellet and ignite nuclear fusion, hovers right around the 1 PW mark, but isn’t the most powerful one around. It has a high amount of energy at over a million joules, but its pulses are, comparatively, very long-duration. To set the power record, you need to deliver the greatest amount of energy in the shortest amount of time.
The current record-holder, instead, uses a sapphire crystal doped with titanium, pumps hundreds of joules of energy into it, bounces the light back-and-forth until destructive interference cancels out most of the pulse length, and the output is compressed into a single pulse just tens of femtoseconds long. That’s how we can reach output powers in the ballpark of 10 PW.
In order to go higher — to reach that next order-of-magnitude milestone — we’ll have to either increase the energy we input into the laser, from hundreds of joules to thousands, or decrease the pulse time. The first one is problematic for the materials we presently use. Small titanium-sapphire crystals won’t hold up to that kind of energy, while larger ones tend to emit light in the wrong direction: at right angles to the desired pathway. The three main approaches, therefore, that researchers are considering at the present time are:
- To take the original, 10 PW pulse, stretch it out over a grating, and combine it into an artificial crystal, where you can pump it again, raising its power.
- To combine multiple pulses from a series of different high-powered lasers to create the right level of overlap: a challenge for pulses just tens of femtoseconds (3–15 microns) long that move at the speed of light.
- Or, to add a second round of pulse compression, squeezing them to as little as a couple of femtoseconds.
The pulses must then be brought to a tight focus, raising not just the power, but the intensity, or the power concentrated at a single point. As the Science article states:
If a 100-PW pulse can be focused to a spot measuring just 3 micrometers across […] the intensity in that tiny area will be an astonishing 1024 watts per square centimeter (W/cm²) — some 25 orders of magnitude, or 10 trillion trillion times, more intense than the sunlight striking Earth.
This opens the door to a long-sought-after opportunity to create particle-antiparticle pairs where there were none before, but it’s hardly “breaking the quantum vacuum.”
According to the theory of quantum electrodynamics, the zero-point energy of empty space isn’t zero, but some positive, finite value. Although we visualize it as particles and antiparticles popping in-and-out of existence, a better depiction is to recognize that, with enough energy, you can — through physics — use these electromagnetic properties of empty space to generate real particle/antiparticle pairs. This is based on the simple Einsteinian physics of E = mc², but requires a strong enough electric field to build those particles: around 10¹⁶ volts per meter. Light, since it’s an electromagnetic wave, carries with it both electric and magnetic fields, and will reach that critical threshold with a laser intensity of 10²⁹ W/cm².
You ought to notice, right away, that even the dream scenario of the science article gives intensities that are still 100,000 times too small to reach this threshold, and whenever you’re below that threshold, your ability to produce particle/antiparticle pairs is exponentially suppressed. The mechanism at play is quite different than simply the reverse of pair production, where instead of an electron and positron annihilating to create two photons, two photons interact to produce an electron/positron pair. (That process was first experimentally demonstrated way back in 1997.) In the laser setup, no individual photons have enough energy to produce new particles, but rather their combined effects on the vacuum of space causes particle/antiparticle pairs to pop into existence with a particular probability. Unless, however, those intensities approach that critical 10²⁹ W/cm² threshold, that probability might as well be zero.
The ability to generate matter/antimatter pairs of particles from empty space alone will be an important test of quantum electrodynamics, and will also be a remarkable demonstration of the power of lasers and our ability to control them. It may not take reaching that critical threshold to generate the first particle/antiparticle pairs from this mechanism, but you’ll have to either get close, get lucky, or have some sort of mechanism to enhance your production over what you naively expect. In any case, the quantum vacuum never breaks, but rather does exactly what you expect of it: responds to matter and energy in accordance with the laws of physics. It might not be intuitive, but it’s something even more powerful: it’s predictable. The art of doing that prediction and doing the experiments to verify or refute them is what science is all about! We may not be there yet, but every leap upwards in power and intensity is another step closer to this “holy grail” in laser physics.
Send in your Ask Ethan questions to startswithabang at gmail dot com!
Ethan Siegel is the author of Beyond the Galaxy and Treknology. You can pre-order his third book, currently in development: the Encyclopaedia Cosmologica.