Ask Ethan: Why Don’t Light And Gravitational Waves Arrive Simultaneously?
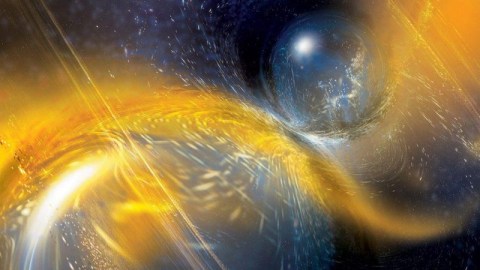
There was less than a 2 second delay between gravitational waves and light, but that’s incredibly meaningful.
There’s an important rule in relativity that — as far as we know — all objects must obey. If you have no rest mass as you travel through the vacuum of space, you absolutely are compelled to travel exactly at the speed of light. This is exactly true for all massless particles, like photons and gluons, approximately true for particles whose mass is tiny compared to their kinetic energy, like neutrinos, and should also be exactly true for gravitational waves. Even if gravity isn’t inherently quantum in nature, the speed of gravity should be exactly equal to the speed of light if our current laws of physics are correct. And yet, when we saw the first neutron star-neutron star merger in both gravitational waves and with light, the gravitational waves got here first by almost 2 seconds. What’s the explanation? That’s what Mario Blanco wants to know, asking:
“I read your articles and found the one on gravitational waves very interesting. […] What would account for the 2s delay of gravitational waves over light waves?”
If everything traveled at the same speed, and both are generated at the same time, then why would one arrive before the other? It’s a great question. Let’s investigate.
On August 17, 2017, the signal from an event that occurred 130 million light-years away finally arrived here on Earth. From somewhere within the distant galaxy NGC 4993, two neutron stars had been locked in a gravitational dance where they orbited one another at speeds that reached a significant fraction of the speed of light. As they orbited, they distorted the fabric of space owing to both their mass and their motion relative to the curved space through which they traveled.
Whenever masses accelerate through curved space, they emit tiny amounts of invisible radiation that’s invisible to all telescopes: gravitational, rather than electromagnetic, radiation. These gravitational waves behave as ripples in the fabric of spacetime, carrying energy away from the system and causing their mutual orbit to decay. At a critical moment in time, these two stellar remnants spiraled so close to one another that they touched, and what followed was one of the most spectacular scientific discoveries of all-time.
As soon as these two stars collided, the gravitational wave signal came to an abrupt end. Everything that the LIGO and Virgo detectors saw was from the inspiral phase up until that moment, followed by total gravitational wave silence. According to our best theoretical models, this was two neutron stars inspiraling and merging together, likely resulting in a remarkable end result: the formation of a black hole.
But then it happened. 1.7 seconds later, after the gravitational wave signal ceased, the first electromagnetic (light) signal arrived: gamma rays, which came in one enormous burst. From the combination of gravitational wave and electromagnetic data, we were able to pin down the location of this event better than any gravitational wave event ever: to the specific host galaxy in which it occurred, NGC 4993.
Over the coming weeks, light began to arrive in other wavelengths as well, as close to 100 professional observatories monitored the spectacular afterglow of this neutron star merger.
On the one hand, this is remarkable. We had an event occur some 130 million light-years away: far enough away that light took 130 million years to travel from the galaxy where it occurred to our eyes. Back when the merger took place, planet Earth was a vastly different place. Feathered birds had been around for only 20 million years; placental mammals for 10 million. The first flowering plants were just beginning to emerge, and the largest dinosaurs were still 30 million years in Earth’s future.
For all that time, from then until the present, both the light and the gravitational waves from this event were journeying through the Universe, traveling at the only speed they could — the speed of light and the speed of gravity, respectively — until they arrived at Earth after a journey of 130 million years. First the gravitational waves from the inspiral phase arrived, moving the mirrors on our gravitational wave detectors by an incredibly small amount: less than a ten-thousandth of the size of an individual proton. And then, just 1.7 seconds after the gravitational wave signal ended, the first light from the event arrived as well.
Immediately, this gave us the most impressive physical measurement of the speed of gravity ever: it was equal to the speed of light to better than 1 part in a quadrillion (1015), as it takes around four quadrillion seconds to make up 130 million years, and they arrived less than two seconds apart from one another. Prior to that, we had excellent theoretical reasons for knowing that the speed of gravity ought to equal the speed of light, but only had indirect constraints that the two were equivalent to within 0.2% or so.
Does this mean that the speed of gravity and the speed of light aren’t quite equal, then? That perhaps either gravity moves slightly faster than c, the speed of light in a vacuum, or that light itself might actually move a tiny bit slower than c, as though it had a tiny but non-zero rest mass to it? That would be an extraordinary revelation, but one that’s highly unlikely. If that were true, light of different energies (and wavelengths) would travel at different speeds, and the level at which that would need to be true is much too large to be consistent with observations.
In simpler terms, if light had a non-zero rest mass, and that mass were heavy enough to explain why gravitational waves arrived 1.7 seconds earlier than light after traveling 130 million light-years across the Universe, then we’d observe radio waves traveling significantly slower than the speed of light: too slow to be consistent with what we’ve already observed.
But that’s okay. In physics, we don’t have any problem considering all possible explanations for an observed puzzle. If we’re doing our jobs correctly, every explanation except for one will be incorrect. The challenge is to find the correct one.
And we think we have! The key is to think about the objects that are merging together, the physics at play, and what signals they’re likely to produce. We’ve already done this for the gravitational waves, detailing how they’re produced during the inspiral phase and cease once the merger takes place. Now, it’s time to go a little deeper and think about the light.
Up until these two neutron stars touched, there was no “extra” light produced. They simply shone as neutron stars do: faintly, at high temperatures but with tiny surface areas, and completely undetectable with our current technology from 130 million light-years away. Neutron stars aren’t like black holes; they aren’t point-like. Instead, they’re compact objects — typically somewhere between 20 and 40 kilometers across — but denser than an atomic nucleus. They’re called neutron stars because they’re about 90% neutrons by composition, with other atomic nuclei and a few electrons at the outer edge.
When two neutron stars collide, there are three possibilities that can result. They are:
- you can form another neutron star, which you’ll do if your total mass is less than 2.5 times the mass of the Sun,
- you can form a new neutron star briefly, which then collapses into a black hole in under a second, if your total mass is between 2.5 and 2.8 solar masses (dependent on the neutron star’s spin),
- or you can form a black hole directly, with no intermediate neutron star, if your total mass is greater than 2.8 solar masses.
From the gravitational wave signal that arose from this event, officially known as GW170817, we know that this event falls into the second category: the merger and post-merger signal existed for a few hundred milliseconds before disappearing entirely all in an instant, which indicates that a neutron star formed for a brief time before an event horizon formed and engulfed the entire thing.
But nevertheless, light still got out. The next question was, simply, how?
How was the light that we observed generated? Again, there were three possibilities that we could think of.
- Immediately, as soon as the neutron stars touch, by processes that occur on their surfaces.
- Only after material gets ejected, where it collides with any surrounding material and produces light from that.
- Or from the interior of neutron stars, where reactions generate energy that only gets emitted once it propagates to the exterior.
In each scenario, gravitational waves travel unperturbed once the signal is generated, but light takes an extra amount of time to get out.
If it’s the first option, and neutron star mergers generate light as soon as they touch, the light gets emitted immediately and therefore must be delayed by passing through the environment surrounding the neutron star. That environment must be rich in matter, as each fast-moving neutron stars, with charged particles on their surfaces and intense magnetic fields, is bound to strip and eject material from the other one.
If it’s the second or third option, merging neutron stars generate light from their mergers, but that light only gets emitted after a certain amount of time has passed: either for ejected material to smash into the circumstellar material or for the light generated in the neutron star interiors to reach the surface. It’s also possible, in either of these cases, that both “delayed emission” and “slowed arrival by surrounding material” are at play.
Any of these scenarios could easily explain the 1.7s delay of light’s arrival with respect to gravitational waves. But on April 25, 2019, we saw another neutron star-neutron star merger in gravitational waves, which was more massive than GW170817. No light was emitted of any type, disfavoring the first scenario. It looks like neutron stars don’t generate light as soon as they touch. Instead, the emission of light comes after the emission of gravitational waves.
With only two direct detections of merging neutron stars through the emission of gravitational waves, it’s a testament to how incredibly precise the science of gravitational wave astronomy has become that we can reconstruct all we have. When you add in the electromagnetic follow-up observations from the 2017 event that also produced light, we’ve definitively shown that a large fraction of the elements in our Universe — including gold, platinum, iodine and uranium — arise from these neutron star mergers.
But not, perhaps, from all neutron star mergers; perhaps it’s only the ones that don’t immediately form a black hole. Either ejected material or reactions in the neutron star’s interior is required to produce these elements, and hence, the light associated with a kilonova explosion. That light is only produced after the gravitational wave signal has ended, and may further be delayed by having to pass through the circumstellar material. This is why, even though light and gravity both travel exactly at the speed of light in a vacuum, the light we saw didn’t arrive until nearly 2 seconds after the gravitational wave signal ceased. As we collect and observe more of these events, we’ll be able to confirm and refine this picture once and for all!
Send in your Ask Ethan questions to startswithabang at gmail dot com!
Ethan Siegel is the author of Beyond the Galaxy and Treknology. You can pre-order his third book, currently in development: the Encyclopaedia Cosmologica.